Doi:10.1016/j.jconhyd.2004.07.002
Journal of Contaminant Hydrology 75 (2004) 281 – 296
Quantifying the effects of fumarate on in situ
reductive dechlorination rates
Kimberly J. Hagemana,*, Jennifer A. Fieldb,
Jonathan D. Istokc, Lewis Semprinic
aDepartment of Chemistry, Oregon State University, 1007 Agriculture and Life Sciences,
Corvallis, OR 97331-7301, United States
bDepartment of Environmental and Molecular Toxicology, Oregon State University,
Corvallis, OR 97331-7301, United States
cDepartment of Civil, Construction, and Environmental Engineering, Oregon State University,
Corvallis, OR 97331-2302, United States
Received 6 August 2003; received in revised form 28 June 2004; accepted 9 July 2004
In situ methods are needed to evaluate the effectiveness of chemical amendments at enhancing
reductive dechlorination rates in groundwater that is contaminated with the priority pollutant,trichloroethene (TCE). In this communication, a method that utilizes single-well, bpush–pullQ tests toquantify the effects of chemical amendments on in situ reductive dechlorination rates is presentedand demonstrated. Five push–pull tests were conducted in each of five monitoring wells located in aTCE-contaminated aquifer at the site of a former chemical manufacturing facility. Rates for thereductive dechlorination of the fluorinated TCE-surrogate, trichlorofluoroethene (TCFE), weremeasured before (test 1) and after (test 5) three successive additions (tests 2–4) of fumarate.
Fumarate was selected to stimulate the growth and activity of indigenous microorganisms with themetabolic capability to reduce TCFE and TCE. In three wells, first-order rate constants for thereductive dechlorination of TCFE increased by 8.2–92 times following fumarate additions. In twowells, reductive dechlorination of TCFE was observed after fumarate additions but not before. Thetransformation behavior of fumarate was also monitored following each fumarate addition.
Correlations between the reductive dechlorination of TCFE and the reduction of fumarate to
* Corresponding author. Tel.: +1 541 737 9862; fax: +1 541 737 0497.
E-mail address:
[email protected] (K.J. Hageman).
0169-7722/$ - see front matter D 2004 Elsevier B.V. All rights reserved.
doi:10.1016/j.jconhyd.2004.07.002
K.J. Hageman et al. / Journal of Contaminant Hydrology 75 (2004) 281–296
succinate were observed, indicating that these reactions were supported by similar biogeochemicalconditions at this site.
D 2004 Elsevier B.V. All rights reserved.
Keywords: Trichloroethene; Reductive dechlorination; Bioremediation; In situ rates; Single-well tests
Significant research efforts have been devoted to the development of in situ
bioremediation as an approach for remediating groundwater contaminated with thepriority pollutant, trichloroethene (TCE). In anaerobic environments, this approachdepends on the metabolic capability of indigenous subsurface microorganisms tocatalyze the reductive dechlorination of TCE to the dichloroethene (DCE) isomers,chloroethene (CE) and ethene ((2000). Engineered approaches are needed where natural attenuation does not result in thecomplete conversion of TCE to ethene or where rates are too slow to meet riskmanagement goals.
A common approach for enhancing in situ reductive dechlorination is to stimulate the
growth of indigenous dechlorinating microorganisms with the addition of chemicalamendments. A wide variety of chemicals and chemical mixtures have been evaluated fortheir suitability as amendments for enhancing reductive dechlorination. reviewed results from laboratory tests that were designed to assess the effectiveness ofpotential amendments such as complex organic mixtures (molasses, wastewater, cheesewhey permeate, corn steep liquor and manure tea), metabolic intermediates (benzoate,lactate, propionate, acetate and butyrate), alcohols (methanol and ethanol), molecularhydrogen, sulfate, nitrate, vitamins and micronutrients. While many of these amendmentswere effective, disadvantages were associated with each and none were universallyeffective at stimulating reductive dechlorination in groundwater at all sites. To the best ofour knowledge, fumarate (trans-1,2-ethenedicarboxylate) has not previously been tested asa chemical amendment for enhancing reductive dechlorination rates. There is evidence thata number of dechlorinating microorganisms use fumarate as an alternative electronacceptor (Gerritse et al., 1996; Krumholz et al., 1996; Krumholz, 1997; Miller et al., 1997; Gerritseet al., 1999) and that certain dechlorinating organisms grow faster on fumarate than onchlorinated ethenes (Some dechlorinating microorganisms are alsoknown to use fumarate (or its reduction product, succinate (1,2-ethanedicarboxylate) (as electron donors during reductivedechlorination. Hence, an objective of this work was to evaluate the effectiveness offumarate at enhancing in situ reductive dechlorination rates.
The effectiveness of a chemical amendment is generally evaluated by comparing
reductive dechlorination rates measured with and without the chemical amendment inlaboratory experiments with pure or mixed cultures of microorganisms. Experimentsconducted in actual aquifers are not as common because field experiments, especially
K.J. Hageman et al. / Journal of Contaminant Hydrology 75 (2004) 281–296
Fig. 1. Analogous reductive dechlorination pathways for (a) TCE and (b) its fluorinated surrogate, TCFE.
those involving well-to-well tests, are perceived as complicated, expensive and/or time-consuming in comparison to laboratory experiments. In addition, in situ transformationrates are difficult to obtain because solute concentrations in groundwater are affected byboth transformation and transport (advection, dispersion and sorption) processes.
Nevertheless, the need for field methods that can be used to evaluate the effectivenessof chemical amendments is becoming increasingly apparent as concerns about discrep-ancies between laboratory and field results mount (1998; Suarez and Rifai, 1999; Washington and Cameron, 2001). Field pilot tests designedto determine in situ reductive dechlorination rates in the presence of chemical amendments(e.g. acetate, nitrate and sulfate) were reviewed by In all but 1 of the 13reported pilot tests, in situ reductive dechlorination rates were determined using well-to-well tests that involved recirculating amended groundwater between injection andextraction wells.
K.J. Hageman et al. / Journal of Contaminant Hydrology 75 (2004) 281–296
An alternative to using well-to-well tests to determine in situ transformation rates is to
use single-well, push–pull tests (1998; Hageman et al., 2001; Schroth et al., 2001; Kleikemper et al., 2002; Pombo et al.,2002). Push–pull tests are conducted by injecting (bpushingQ) an aqueous test solutioncontaining a nonsorbing, nonreactive tracer and one or more reactants into the saturatedzone of an aquifer via a monitoring well. Samples of the test solution/groundwatermixture are then extracted (bpulledQ) from the same well over time and analyzed fortracer, reactant and product concentrations. The in situ transformation rate of an injectedreactant is then determined by removing the effects of transport processes from measuredreactant concentrations using a data processing technique. Push–pull tests are cost-effective relative to well-to-well tests because push–pull tests require only onegroundwater well per test. Additional advantages are that push–pull tests take less timeto conduct than well-to-well tests since injected solutes do not have to be transportedbetween wells and that push–pull tests can be conducted simultaneously in differentwells to assess spatial variability in transformation rates. described the development of push–pull tests for determining in situ reductivedechlorination rates and demonstrated the technology by conducting push–pull tests ina TCE-contaminated aquifer. The injected test solution contained trichlorofluoroethene(TCFE), which was used as a surrogate for TCE based on evidence that it undergoesreductive dechlorination by a pathway analogous to that of TCE while retaining thefluorine label ((TCE itself was not injected intoTCE-contaminated groundwater because mixing of the injected test solution with nativegroundwater would have rendered it impossible to distinguish injected and backgroundTCE.
The overall objective of the project described herein was to use the approach presented
by to quantify the effects of fumarate additions on in situ reductivedechlorination rates of TCFE. To this end, TCFE reductive dechlorination rates weremeasured before and after three consecutive additions of fumarate in five wells located in aTCE-contaminated aquifer. Additionally, the transformation behavior of fumarate wasmonitored after each fumarate addition so that correlations between reductive dechlori-nation and fumarate transformation behavior could be assessed.
TCFE (97% pure, containing 0.1% cis-DCFE and 0.3% trans-DCFE), cis/trans-1,2-
dichloroethene (DCFE) (98%) and E/Z-1-chloro-2-fluoroethene (97%) were obtainedfrom SynQuest Laboratories (Alachua, FL). Fluoroethene (FE) (98%) was obtainedfrom Lancaster Synthesis (Pelham, NH). Sodium fumarate (98%) and sodium succinate(99%) were obtained from Aldrich (Milwaukee, WI). Potassium bromide (99.7%) andsodium formate (99.6%) were obtained from Fisher Scientific (Fair Lawn, NJ). For useas an internal standard, 1-chloropropane was obtained from Matheson (Cincinnati,OH).
K.J. Hageman et al. / Journal of Contaminant Hydrology 75 (2004) 281–296
Table 1Biogeochemical indicator concentrations in tested wells
Concentration (AM)a
Total organic carbon
Total dissolved iron
a Samples were collected in May and June 1999. Total organic carbon by EPA 9060, dissolved oxygen by
membrane electrode probe, total dissolved iron by EPA 6010B, nitrate and sulfate by EPA 9056, and methane byRSK-175.
b Not detected.
2.2. Site description
Push–pull tests were conducted at the site of a former chemical manufacturing facility
near San Francisco, CA where reductive dechlorination has been monitored in recent years(Tests were conducted intwo distinct aquifer zones. The A-zone is an unconfined shallow layer composed mainly ofplaced fill over Bay Mud. The water table lies within 3 meters of the ground surface.
Groundwater velocities range from 1.5 to 6 m/year. The C-zone underlies the Bay Mud, ischaracterized by alluvial fan deposits, and is located approximately 6–23 m below theground surface. Groundwater velocities range from 6 to 31 m/year. Biogeochemicalindicator concentrations for each well are given in
2.3. Push–pull tests
A series of five push–pull tests were conducted in each of two A-zone wells (10A and
9A) and in each of three C-zone wells (15C, 16C and 21C) between December 1999 andFebruary 2001 (All test solutions contained 1.3 mM of bromide, which servedas a conservative tracer. All test solutions injected during test 1, which was designed to
Table 2Test solution compositions
K.J. Hageman et al. / Journal of Contaminant Hydrology 75 (2004) 281–296
measure initial rates for TCFE reductive dechlorination, contained TCFE (Additionally, fumarate was included in the test solution injected into well 9A. While thefocus of this project was not to measure the effects of formate on reductive dechlorinationrates, formate was included in test solutions injected into wells 15C and 16C becauseprevious measurements indicated that electron donors in the C-zone may limit reductivedechlorination rates. Rates obtained during test 1 in wells 10A, 15C and 21C werepreviously reported (however, they differ from the rates reportedherein because a different data processing techniques was used. The objectives of tests 2–4were to amend the groundwater with fumarate and then to monitor the fumaratetransformation behavior following each addition. Thus, test solutions injected during tests2–4 contained fumarate (The objective of test 5 was to measure reductivedechlorination rates after the fumarate additions so that rate changes due to fumarate couldbe quantified. Therefore, test solutions injected during test 5 contained TCFE, fumarateand formate at concentrations similar to those used in test 1 (
The experimental design was identical to that described in
where a diagram of the experimental set-up and further information can be found. Testsolutions were prepared on-site by adding bromide and fumarate or formate (toargon-sparged tap water. In tests 1 and 5, a concentrated aqueous solution of TCFE wasmetered into the injection line. The TCFE solution was stored and pumped from acollapsible metallized-film gas-sampling bag to prevent volatilization loss duringinjection. In A-zone wells, which were 2.5 cm in diameter, 25–50 l of test solution wereinjected into the bottom of the well at a rate of 0.2 l/min. In C-zone wells, which were 10cm in diameter and capable of accepting a greater injection flow rate, 250 l of testsolution were injected at a rate of 2 l/min between a pair of inflatable packers used toisolate a meter-long section of the well screen. Following the injection, samples of the testsolution/groundwater mixture were collected approximately once per week for up to 85days during tests 1 and 5 and on a varying schedule, with a maximum of four samples perday, for up to 20 days during tests 2–4. Samples were collected by extracting water with aperistaltic pump from the bottom of A-zone wells or from between the inflatable packers inC-zone wells. Volatile organic analysis vials (40 ml) were filled without headspace afterpurging A-zone and C-zone wells of 0.3 and 12 l of water, respectively. Samples werecollected in duplicate or triplicate, shipped on ice and stored at 4 8C until analysis. Allsamples except those for bromide were preserved in 0.75% (v/v) concentrated HCl.
Duplicate analyses were preformed on 10% of samples.
2.4. Analytical methods
Chromatographic separation and detection of TCFE, DCFE, CFE and FE were
achieved using a gas chromatography/mass spectrometry method that was previouslydescribed (The analyte introduction method was either headspaceanalysis (test 1 samples) or purge-and-trap (test 5 samples). The purge-and-trap systemwas composed of a Tekmar-Dohrmann (Cincinnati, OH) 3100 sample concentrator and anAQUA Tek 70 liquid analyzer. Quantification was performed in selected ion monitoringmode with 1-chloropropane as an internal standard. Chromatographic separation anddetection of organic acids in test series 1–4 samples were performed on a Waters Alliance
K.J. Hageman et al. / Journal of Contaminant Hydrology 75 (2004) 281–296
(Milford, MA) high performance liquid chromatograph equipped with a photodiode arraydetector. Separations were achieved on a Phenomenex (Torrance, CA) Luna C18 column.
Test series 5 samples were analyzed on a Dionex (Sunnyvale, CA) DX-320 ionchromatograph equipped with a conductivity detector and AS11 column. Bromideconcentrations were measured with either a Dionex DX-120 or Dionex DX-320 ionchromatograph equipped with a conductivity detector and Dionex AS14 or AS11 column.
2.5. Data analysis
In situ rates for the reductive dechlorination of TCFE were determined by removing the
effects of transport processes from measured aqueous concentrations of TCFE using a dataprocessing technique called bforced mass balanceQ (FMB) (TCFEtransformation products were also treated with the FMB technique so that the distributionof transformation products formed in situ could be readily compared between tests. FMBwas selected over other available data processing techniques (Snodgrass and Kitanidis, 1998) because it was designed for use with sorbing solutes.
TCFE and its products were expected to undergo sorption during push–pull tests in bothA- and C-zones based on results from short-term transport tests conducted at the selectedsite (as well as estimated retardation factor (R) values.
The FMB technique (was conducted by first calculating total
(aqueous plus sorbed) concentrations for TCFE and each transformation product bymultiplying the respective R values for each solute by their molar aqueousconcentrations measured in push–pull test extraction samples. The R values estimatedfor TCFE, DCFE, CFE and FE were 11.5, 4.89, 2.41 and 1.69, respectively, in the A-zone and 2.05, 1.39, 1.14 and 1.07, respectively, in the C-zone. These values wereestimated using Kom values for TCFE, DCFE, CFE and FE of 90.5,33.5, 12.2 and 5.99 l/kg, respectively; a bulk density of 2.32 kg/l; an aquifer porosity of0.2; and fraction organic matter values of 0.01 (A-zone) and 0.001 (C-zone). The Komvalues were estimated using the Estimations Program Interface Suite (2000), while the other values were selected based on measurements made on aquifersediments collected at the site.
A btransport-processQ adjustment factor (
o are the sums of the
total concentrations of TCFE and its transformation products in the extraction sample andin the well at the end of the test solution injection, respectively) was then calculated for
each extraction sample. Next, total concentrations were divided by corresponding
values to remove the effects of transport processes (including sorption) and obtain FMB-adjusted concentrations. Finally, nonlinear least squares analysis was used to derive theequation that best described changes in FMB-adjusted concentrations of TCFE over time.
Reviews of reported half-velocity coefficients for reductive dechlorination indicate that itis appropriate to assume first-order kinetics when chlorinated ethene concentrations areseveral AM or lower (Since allmeasured aqueous concentration of TCFE were less than 2 AM except for those measuredin the first two extraction samples collected in wells 10A and 21C, TCFE transformationwas assumed to be governed by first-order kinetics. Based on evidence that sorbed-phasereactants are not bioavailable (it was assumed that only TCFE in the
K.J. Hageman et al. / Journal of Contaminant Hydrology 75 (2004) 281–296
aqueous phase (and not in the sorbed phase) underwent transformation. Thus, equationsused to describe changes in FMB-adjusted concentrations of TCFE were of the form
expð kt=RðTCFEÞÞ
where [TCFE]FMB,o is the FMB-adjusted concentration of TCFE in the well at the end ofthe test solution injection, k is the first-order rate constant describing the rate of change intotal concentrations of TCFE due to transformation alone, t is time and R(TCFE) is theretardation factor for TCFE. The validity of the FMB technique was evaluated byquantifying errors in rates derived by applying FMB to push–pull test data generated by anumerical model (Since the error analysis indicated that the in siturate for the reductive dechlorination of TCFE was underestimated relative to the actual insitu rate by 10%, all rates reported herein are expected to be underestimated by a similarmagnitude.
The effects of transport processes were removed from measured aqueous concentrations
of fumarate, succinate and formate using a data processing technique developed for usewith nonsorbing solutes that is referred to herein as btracer-normalizationQ (1998; Snodgrass and Kitanidis, 1998). Sorption was assumed to have a minimal effect onfumarate, succinate and formate concentrations since these solutes are negatively chargedand highly water-soluble. Tracer-normalized concentrations for each solute were obtainedby dividing their measured aqueous concentrations by the transport-process adjustmentfactor ([Br]/[Br]o, where [Br] and [Br]o are the measured concentrations of the co-injectedbromide tracer in an extraction sample and in the injected test solution, respectively).
3. Results and discussion
3.1. Reductive dechlorination rates and product distribution ratios
Reductive dechlorination of TCFE occurred following its first injection into well 10A
(test 1) as indicated by decreasing aqueous TCFE concentrations and increasing aqueouscis-DCFE concentrations (Trans-DCFE and (E)-CFE were also detected atrelatively low concentrations (b0.05 AM). FMB-adjusted concentrations were calculatedfor each solute (see Section 2.5) (The exponential equation that best described thechange in FMB-adjusted concentrations of TCFE over time,
¼ 180lM expð 0:012t=11:5Þ;
indicated that the in situ rate (k) for the transformation of TCFE to DCFE was 0.012 day1(FMB-adjusted concentrations for TCFE and its transformation productsobtained for test 1 in well 10A were also plotted in a stacked area graph (tofacilitate visualization of TCFE/product distribution ratios.
After three successive additions of fumarate (tests 2–4), which were made 1–2 months
apart, TCFE was injected into well 10A for a second time (test 5). Reductivedechlorination of TCFE to cis-DCFE, trans-DCFE, CFE (EN1,1NZ) and FE occurred(with TCFE being transformed at a maximum rate of 1.1 day1 between days 30
K.J. Hageman et al. / Journal of Contaminant Hydrology 75 (2004) 281–296
Fig. 2. Test 1 in well 10A. (a) Measured aqueous concentrations indicating concentration changes due totransformation and transport processes (with expanded view in inset) and (b) forced mass balance (FMB)-adjustedconcentrations (see data analysis section) indicating concentration changes due to transformation only.
and 84 (Thus, the maximum transformation rate was 92 times greater afterfumarate additions than before. Moreover, the extent of dechlorination increased, asindicated by detection of the less chlorinated products. The formation of FE had importantimplications for bioremediation at this site because it is the fluorinated analog of ethene((and ethene is the desired end product for reductivedechlorination due to its low toxicity.
Following the first injection of TCFE into well 9A (test 1), TCFE underwent reductive
dechlorination primarily to cis-DCFE although trans-DCFE was also formed (The rate of TCFE transformation was 0.021 day1 (Note that fumarate was co-injected with TCFE during the initial test conducted in well 9A but not during the initialtest conducted in well 10A. After three additional injections of fumarate (tests 2–4) to well9A, TCFE and fumarate were co-injected into well 9A for a second time (test 5). TCFEunderwent reductive dechlorination primarily to cis-DCFE although trans-DCFE and CFE(EN1,1NZ) were also formed (TCFE was transformed at a maximum rate of 1.6day1 between days 7 and 50 (Thus, the maximum transformation rate was 76times greater during test 5 than during test 1. While TCFE transformation rates obtained
Table 3TCFE transformation rates
Maximum first-order rate constants for transformation of TCFE to DCFE (day1)a
0.012 (0–80 days)
1.1 (30–84 days)
0.021 (0–82 days)
1.6 (7–50 days)
0.017 (55–82 days)
0.14 (0–84 days)
0.051 (49–84 days)
0.15 (16–84 days)
a Transformation rates were calculated from FMB-adjusted concentrations over the period of days shown in
parenthesis. Reported rates are expected to be underestimated relative to actual rates by 10% based on resultsfrom a computer modeling study ((see Section 2.5).
b Transformation of TCFE to DCFE was not observed.
K.J. Hageman et al. / Journal of Contaminant Hydrology 75 (2004) 281–296
Fig. 3. Stacked area plots of FMB concentrations depicting reductive dechlorination of TCFE during testsconducted before (test 1) and after (test 5) fumarate additions in A-zone wells.
during test 5 in wells 10A and 9A were similar, the extent of dechlorination observed inthese wells was remarkably different. In well 9A, cis-DCFE was formed almostexclusively (while in well 10A, the dechlorination of TCFE to FE was observed(). This indicates that the microbial populations available for stimulation byfumarate were different in the two wells even though the wells were located within 25 m ofeach other and in the same horizontal subsurface zone.
Significant reductive dechlorination was not observed until 55 days after the first
injection of TCFE into well 15C (test 1) (At that point, TCFE underwentreductive dechlorination primarily to cis-DCFE although trans-DCFE was also formed.
TCFE was transformed at a rate of 0.017 day1 between days 55 and 82 (Toincrease the likelihood that reductive dechlorination would occur, formate was co-injectedas an electron donor with TCFE during this test. Co-injected formate was completelydegraded by day 27 (data not shown), indicating that an active community of formate-utilizing organisms was present. However, acetate, which would have been formed duringacetogenesis of injected formate, was not detected. After three successive injections offumarate (tests 2–4), TCFE and formate were co-injected into well 15C for a second time(test 5). Reductive dechlorination of TCFE to primarily cis-DCFE and CFE (Ec1,1NZ)occurred (although trans-DCFE was also formed. TCFE was transformed at amaximum rate of 0.14 day1 between days 0 and 84 (Thus, the maximum
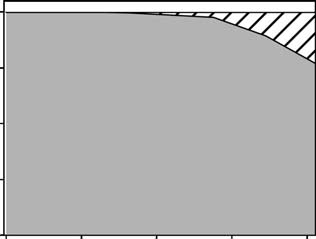
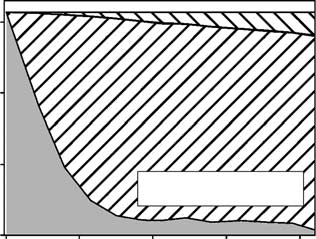
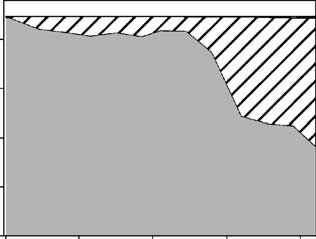
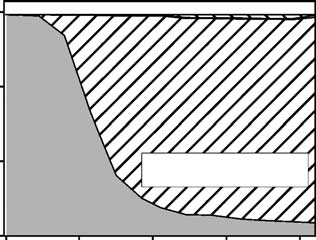
K.J. Hageman et al. / Journal of Contaminant Hydrology 75 (2004) 281–296
Fig. 4. Stacked area plots of FMB concentrations depicting reductive dechlorination of TCFE or lack thereofduring test conducted before (test 1) and after (test 5) fumarate additions in C-zone wells.
transformation rate was 8.2 times greater after fumarate additions than before. However,it may be of greater significance that the transformation reaction began immediately duringtest 5 instead of being delayed until late in the test as it was during test 1. Co-injectedformate was not detected in any samples, indicating that the formate-utilization rate alsoincreased between tests. This observation is consistent with the expectation that electron-donor utilization and reductive dechlorination rates should increase together. Again,acetate was not detected. Note that because formate was added as an amendment withTCFE in tests 1 and 5, the project was not designed to evaluate the effect of formate onreductive dechlorination rates.
K.J. Hageman et al. / Journal of Contaminant Hydrology 75 (2004) 281–296
In contrast to what was observed in all previously described wells, reductive
dechlorination was not observed after the first injection of TCFE into well 16C (test 1)(Formate, which was again co-injected as an electron donor with TCFE,underwent degradation slowly such that it was still detected in samples collected at the endof the test (data not shown). This observation is consistent with the expectation that a slowformate-utilization rate would accompany a slow or non-detectable TCFE reductivedechlorination rate. Acetate formation via the potential acetogenesis pathway was notdetected. After three successive additions of fumarate (tests 2–4), TCFE and formate wereco-injected into well 16C for a second time (test 5). Reductive dechlorination of TCFEprimarily to cis-DCFE occurred although trans-DCFE and CFE (Ec1,1NZ) were alsoformed (TCFE was transformed at a maximum rate of 0.051 day1 between days49 and 84 (Co-injected formate was not detected, indicating that formate-utilization and TCFE reductive dechlorination rates increased together, as was observed inwell 15C. Although relatively high concentrations of TCFE persisted until the end of thetest, it is of particular significance that TCFE reductive dechlorination was stimulated (test5) (in a well where it had not initially been observed (test 1) (
Well 21C is located upgradient from the contaminant plume in an uncontaminated
location. Reductive dechlorination of TCFE was not observed following the first injectionof TCFE (test 1) (which is consistent with the expectation that dechlorinatingmicroorganisms would not be active in the absence of chlorinated compounds. After threesuccessive additions of fumarate (tests 2–4), TCFE was injected for a second time (test 5).
Reductive dechlorination of TCFE primarily to cis-DCFE occurred although trans-DCFEand CFE (ENN1,1cZ) were also formed (TCFE was transformed at a maximumrate of 0.15 day1 between days 16 and 84 (The occurrence of TCFE reductivedechlorination in well 21C during test 5 is significant because it indicates that reductivedechlorination was stimulated in an uncontaminated well.
3.2. Correlations between fumarate and TCFE transformation behavior
Three types of fumarate transformation behavior were observed during tests 2–4. The
first type of behavior is classified as that in which fumarate was reduced to succinate uponthe first fumarate injection and the rate at which fumarate was reduced to succinateincreased upon each additional fumarate injection. This type of behavior was observed inwells 10A and 9A. In well 10A, for example, fumarate concentrations decreased moreslowly in test 2 than in test 4 (Likewise, succinate formation was observed later intest 2 than in test 4. In both tests 2 and 4, succinate concentrations decreased toundetectable levels, indicating that microbial populations utilized succinate. Based onresults from laboratory experiments (it is likely that succinate wasutilized as an electron donor. In wells 10A and 9A, the transformation behavior exhibitedby TCFE was similar to that exhibited by fumarate in that reductive dechlorination ratesincreased between tests 1 and 5. The similarities between TCFE and fumaratetransformation behaviors indicate that reductive dechlorination and fumarate reductionmay be supported by similar biogeochemical conditions in the A-zone. Note that, byplotting tracer-normalized concentrations in changes in concentration due only totransformation are depicted (see Section 2.5); however, in contrast to what was observed in
K.J. Hageman et al. / Journal of Contaminant Hydrology 75 (2004) 281–296
Fig. 5. Tracer-normalized (TN) concentrations (see Section 2.5) of fumarate and its reduction product, succinate.
for aqueous and FMB concentrations, aqueous and tracer-normalized concentrationswere not significantly different. For example, on day 1 during test 4 in well 10A, measuredaqueous concentrations of fumarate and succinate were 0.35 and 0.20 mM, respectively,while corresponding tracer-normalized concentrations were 0.37 mM and 0.21 mM.
The second type of fumarate transformation behavior is classified as that in which the
reduction of fumarate to succinate was not observed after its first addition (test 2) but wasobserved after later additions (e.g. test 4). This type of behavior was observed in wells 15Cand 21C. In well 21C, for example, fumarate concentrations decreased without theconcomitant detection of succinate in test 2 (however, decreases in fumarateconcentrations were accompanied by succinate detection in test 4. While it is possible thatsuccinate was not detected because it was rapidly utilized, the detection of succinate in test4 indicates that it is likely that microbial populations capable of reducing fumarate tosuccinate were stimulated by successive fumarate additions. The TCFE transformationbehavior observed in wells 15C and 21C was consistent with that observed for fumaratesince TCFE reductive dechlorination was either observed only after a significant lag timeor not observed at all in these wells during the first TCFE injection (test 1). Yet, TCFEreductive dechlorination was observed in these wells during the second TCFE injection(test 5). This correlative behavior indicates that reductive dechlorination and fumaratereduction may be supported by similar biogeochemical conditions in the C-zone.
The third type of fumarate transformation behavior is classified as that in which the
reduction of fumarate to succinate was not observed during any of the fumarate additions
K.J. Hageman et al. / Journal of Contaminant Hydrology 75 (2004) 281–296
(tests 2–4). This type of behavior was observed in well 16C only (The hypothesisthat reductive dechlorination and fumarate reduction were supported by similarbiogeochemical conditions is further supported by observations in well 16C since thereductive dechlorination rate obtained during test 5 in well 16C was lower than thatobtained in any other well during test 5 (
Although TCFE and fumarate displayed parallel transformation behaviors in all five
wells at this site, further investigation is needed to determine what processes wereresponsible for increases in TCFE reductive dechlorination rates. An importantconsideration, however, is that in all wells (except well 16C) both injected fumarateand succinate (formed in situ) were degraded to undetectable concentrations within 10days after each fumarate injection (tests 2–4) (Since neither fumarate nor succinatewas present in the groundwater during the final TCFE injection (test 5), it was not thepresence of either of these compounds that caused reductive dechlorination rates toincrease. Rather, the consecutive additions of fumarate appeared to have altered thebiogeochemical conditions in a way that favored reductive dechlorination. Since previousreports indicate that a number of dechlorinating microorganisms utilize fumarate as analternative electron acceptor (Gerritse et al., 1996, 1999; Krumholz et al., 1996; Krumholz, 1997; Miller et al., 1997)and that at least one of them grows faster on fumarate than on chlorinated ethenes (et al., 1999), it is possible that fumarate additions stimulated the growth of microbialpopulations that utilize both fumarate and chlorinated ethenes. Therefore, a change inmicrobial community structure could have been responsible for the enhancement ofreduction rates for both TCFE and fumarate. However, it is also possible that changes inmicrobial populations were induced by the presence of succinate (formed in situ), whichcan be used as an electron donor (or by fumarate acting as anelectron donor instead of as an electron acceptor (Note thatbackground total organic carbon, which includes compounds that can potentially serve asnatural electron donors, was present at a higher concentration than injected fumarate (1.3mM) in well 10A, at a similar concentration in well 9A, and was not detected in C-zonewells (Other potential scenarios are that fumarate acted as an electron donor toreduce the concentrations of indigenous electron acceptors or that TCFE itself stimulatingthe growth of dechlorinating microorganisms. On-going research in our group with soilmicrocosms is designed to further characterize the relation between fumarate and TCE/TCFE reductive dechlorination rates.
This communication describes the application of a methodology for quantifying
changes in in situ reductive dechlorination rates due to the addition of chemicalamendments. This methodology has the potential to improve bioremediation technologiesbecause it can be used to measure the effectiveness of bioremediation technologies in thefield. To the best of our knowledge, this communication also describes the first use offumarate in a field method designed to enhance reductive dechlorination rates. TCFEreductive dechlorination rates increased from 8.2 to 92 times in wells where reductive
K.J. Hageman et al. / Journal of Contaminant Hydrology 75 (2004) 281–296
dechlorination was initially observed. Reductive dechlorination activity was stimulated inwells where it was not initially observed. Similarities in the transformation behaviors ofTCFE and fumarate indicated that reductive dechlorination and fumarate reduction weresupported by similar biogeochemical conditions.
We thank Timothy Buscheck, Kirk O'Reilly, Ralph Reed and Mark Dolan for their
contributions. Kevin Tam, Robert Alumbaugh, Brian Davis, Jesse Jones and AngelitoTirona assisted in the field. This project was funded in part by grant number 1P42ES10338 from the National Institute of Environmental Health Sciences (NIEHS), withfunds from the U.S. Environmental Protection Agency. Kimberly Hageman was supportedby a training core grant from NIEHS 1P42 ES10338. The Western Region HazardousSubstance Research Center and the ChevronTexaco Energy Research and Technologyprovided additional support.
Bradley, P.M., 2000. Microbial degradation of chloroethenes in groundwater systems. Hydrogeol. J. 8 (1),
104 – 111.
Buscheck, T.E., 1998. Intrinsic anaerobic biotransformation of chlorinated solvents at a manufacturing
plant. Chevron Research and Technology Unpublished Report, Chevron Research and Technology,Richmond, CA.
Buscheck, T.E., O'Reilly, K.T., Hickman, G., 1997. Intrinsic anaerobic biodegradation of chlorinated solvents at a
manufacturing plant. In: Leeson, A., Alleman, B.C. (Eds.), In Situ and On-Site Bioremediation. Battelle Press,Columbus, OH, pp. 149 – 154.
Chapelle, F.H., Lovely, D.R., 1990. Rates of microbial metabolism in deep coastal plain aquifers. Appl. Environ.
Microbiol. 56 (6), 1865 – 1874.
Gerritse, J., Renard, V., Gomes, T.M.P., Lawson, P.A., Collins, M.D., Gottschal, J.C., 1996. Desulfitobacterium
sp. strain PCE1, an anaerobic bacterium that can grow by reductive dechlorination of tetrachloroethene orortho-chlorinated phenols. Arch. Microbiol. 165, 132 – 140.
Gerritse, J., Drzyzga, O., Kloetstra, G., Keijmel, M., Wiersum, L.P., Hutson, R., Collins, M.D., Gottschal, J.C.,
1999. Influence of different electron donors and acceptors on dehalorespiration of tetrachloroethene byDesulfitobacterium frappieri TCE1. Appl. Environ. Microbiol. 65 (12), 5212 – 5221.
Hageman, K.J., Istok, J.D., Field, J.A., Buscheck, T.E., Semprini, L., 2001. In situ anaerobic transformation
of trichlorofluoroethene in trichloroethene-contaminated groundwater. Environ. Sci. Technol. 35 (9),1729 – 1735.
Hageman, K.J., Field, J.A., Istok, J.D., Schroth, M.H., 2003. bForced mass balanceQ technique for estimating in
situ transformation rates of sorbing solutes in groundwater. Environ. Sci. Technol. 37, 3920 – 3925.
Haggerty, R., Schroth, M.H., Istok, J.D., 1998. Simplified method of bpush–pullQ test data analysis for
determining in situ reaction rate coefficients. Ground Water 36 (2), 314 – 324.
Haston, Z.C., McCarty, P.L., 1999. Chlorinated ethene half-velocity coefficients (Ks) for reductive dechlorination.
Environ. Sci. Technol. 33 (2), 223 – 226.
Istok, J.D., Humphrey, M.D., Schroth, M.H., Hyman, M.R., O'Reilly, K.T., 1997. Single-well, bpush–pullQ test
method for in situ determination of microbial metabolic activities. Ground Water 35 (4), 619 – 631.
Kengen, S.W.M., Breidenbach, C.G., Felske, A., Stams, A.J.M., Schraa, G., Vos, W.M.d., 1999. Reductive
dechlorination of tetrachloroethene to cis-1,2-dichloroethene by a thermophilic anaerobic enrichment culture.
Appl. Environ. Microbiol. 65 (6), 2313 – 2316.
K.J. Hageman et al. / Journal of Contaminant Hydrology 75 (2004) 281–296
Kleikemper, J., Schroth, M.H., Sigler, W.V., Schmucki, M., Bernasconi, S.M., Zeyer, J., 2002. Activity and
diversity of sulfate-reducing bacteria in a petroleum hydrocarbon-contaminated aquifer. Appl. Environ.
Microbiol. 68 (4), 1516 – 1523.
Krumholz, L.R., 1997. Desulfuromonas chloroethenica sp. nov. uses tetrachloroethylene and trichloroethylene as
electron acceptors. Int. J. Syst. Bacteriol. 47 (4), 1262 – 1263.
Krumholz, L.R., Sharp, R., Fishbain, S.S., 1996. A freshwater anaerobe coupling acetate oxidation to
tetrachloroethylene dehalogenation. Appl. Environ. Microbiol. 62 (11), 4108 – 4113.
Lee, M.D., Quinton, G.E., Beeman, R.E., Biehle, A.A., Liddle, R.L., Ellis, D.E., Buchanan Jr., R.J., 1997. Scale-
up issues for in situ anaerobic tetrachloroethene bioremediation. J. Ind. Microbiol. Biotech. 18, 106 – 115.
Lee, M.D., Odom, J.M., Buchanan, R.J.J., 1998. New perspectives on microbial dehalogenation of chlorinated
solvents: insights from the field. Annu. Rev. Microbiol. 52, 423 – 452.
Lyman, W., Reidy, P.J., Levy, B., 1992. Mobility and Degradation of Organic Contaminants in Subsurface
Environments. CK Smoley, Chelsea, MI.
McCarty, P.L., 1997. Breathing with chlorinated solvents. Science 276 (5318), 1521.
Miller, E., Wohlfarth, G., Diekert, G., 1997. Comparative studies on tetrachloroethene reductive dechlorination
mediated by Desulfitobacterium sp. strain PCE-S. Arch. Microbiol. 168, 513 – 519.
Neumann, A., Scholz-Muramatsu, H., Diekert, G., 1994. Tetrachloroethene metabolism of Dehalospirillum
multivorans. Arch. Microbiol. 162, 295 – 301.
Neumann, A., Wohlfarth, G., Diekert, G., 1995. Properties of tetrachloroethene and trichloroethene dehalogenase
of Dehalospirillum multivorans. Arch. Microbiol. 163, 276 – 281.
Pombo, S.A., Pelz, O., Schroth, M.H., Zeyer, J., 2002. Field scale 13-C-labeling of phospholipid fatty acids
(PLFA) and dissolved inorganic carbon: tracing acetate assimilation and mineralization in a petroleumhydrocarbon-contaminated aquifer. FEMS Microbiol. Ecol. 41, 259 – 267.
Schnoor, J.L., 1996. Environmental Modeling: Fate and Transport of Pollutants in Water, Air, and Soil. John
Wiley and Sons, New York.
Scholz-Muramatsu, H., Neumann, A., Megmer, M., Moore, E., Diekert, G., 1995. Isolation and characterization
of Dehalospirillum multivorans gen. nov., a tetrachloroethene-utilizing, strictly anaerobic bacterium. Arch.
Microbiol. 163, 48 – 56.
Schroth, M.H., Istok, J.D., Conner, G.T., Hyman, M.R., Haggerty, R., O'Reilly, K.T., 1998. Spatial variability in
in situ aerobic respiration and denitrification rates in a petroleum-contaminated aquifer. Ground Water 36 (6),924 – 937.
Schroth, M.H., Kleikemper, J., Bollinger, C., Bernasconi, S.M., Zeyer, J., 2001. In situ assessment of microbial
sulfate reduction in a petroleum-contaminated aquifer using push–pull tests and stable sulfur isotope analysis.
J. Contam. Hydrol. 51 (3–4), 179 – 195.
Snodgrass, M.F., Kitanidis, P.K., 1998. A method to infer in situ reaction rates from push–pull experiments.
Ground Water 36 (4), 645 – 650.
Suarez, M.P., Rifai, H.S., 1999. Biodegradation rates for fuel hydrocarbons and chlorinated solvents in
groundwater. Biorem. J. 3 (4), 337 – 362.
Syracuse Research, 2000. Estimations Programs Interface Suite.
U.S. EPA, 1998. Technical Protocol for Evaluating Natural Attenuation of Chlorinated Solvents in Ground Water.
EPA/600/R-98/128, Cincinnati, OH.
Vancheeswaran, S., Hyman, M.R., Semprini, L., 1999. Anaerobic bio-transformation of trichlorofluoroethene
(TCFE) in groundwater microcosms. Environ. Sci. Technol. 33, 2040 – 2045.
Vogel, T.M., Criddle, C.S., McCarty, P.L., 1987. Transformations of halogenated aliphatic compounds. Environ.
Sci. Technol. 21, 722 – 736.
Washington, J.W., Cameron, B.A., 2001. Evaluating degradation rates of chlorinated organics in groundwater
using analytical models. Environ. Toxicol. Chem. 20 (9), 1909 – 1915.
Source: http://wrhsrc.oregonstate.edu/semprini/pub_pdfs/jcontamhydrology.pdf
Formulary decision guide: Dexamethasone 2 mg/5 ml oral solution (Dexsol®) Product current status: • More severe croup (or mild croup that might cause complications) calls for hospital admission:5 Product proposed status: – a single dose of corticosteroid should be administered Date of next meeting: before transfer to hospital
Vol 23, Number 1 Journal of Forensic Medicine, Science and Law (Jan-Jun 2014) A Journal of Medicolegal Association of Maharashtra Case Report DEATHS DUE TO CHOKING AMONG PSYCHIATRIC PATIENTS A REPORT OF THREE CASES Dr. CV Tingne, Dr. NB Kumar, Dr. PS Ghormade, Dr. RK Gadhari, Dr. AN Keoliya Dr. Chaitanya Vidyadhar Tingne Assistant Professor, Department of Forensic Medicine & Toxicology, Indira Gandhi Government Medical College, Nagpur, Maharashtra, India (440018) Dr. Narendra Baluram Kumar Assistant Professor, Department of Forensic Medicine & Toxicology, Indira Gandhi Government Medical College, Nagpur, Maharashtra, India (440018)