Spinal sensorimotor system part ii:
SSMS II: Neurons
Spinal Sensorimotor System Part II:
Motoneurons and Motoneuron Pathways
Classes of Neurons and Afferents
The standard way of classifying neurons in the spinal cord and dorsal root ganglion leaves a
bit to be desired in the way of crispness, but by and large classifications are based on where the neuron receives its input and where it projects its output. There are three major categories:
1. Afferent neurons (also called extra-spinal-cord neurons):
These are the sensory neurons. They consist of the spindle sensor neurons (the group Ia, Ib, II, III, and IV motor afferents), the joint sensor neurons (types I, II, III, IV joint afferents), and the cutaneous skin receptor neurons (typically divided up as high-threshold and low-threshold afferents, sometimes divided up as nociceptors and mechanico-sensors).
These are the neurons that actually drive the muscle cells. There are five classes, designated as alpha (a-MNs), static beta (bs-MNs), dynamic beta (bd-MNs), static gamma (gs-MNs), and
dynamic gamma (gd-MNs). The a-MNs are the main drive neurons for the extrafusal muscle
fibers, and hence are known as slow (S), fast-resistant to fatigue (FR), and fast-fatiguing (FF)
skeleto-motoneurons. The b-MNs are actually small a-MNs with very small-diameter axons.
To date they have not been studied extensively, but it is believed that they share many of the same types of inputs as the a-MNs.he innervation of the muscle by b-MNs was illustrated
in figure 6 of the "Muscles" tech brief. In recognition of their connection to both extrafusal and
intrafusal fibers, they are known as
skeleto-fusimotor neurons. The g-MNs innervate the
intrafusal spindle fibers, thereby exercising direct control on the biasing and sensitivity of the
muscle afferent sensors of groups Ia and II. Their connection to muscle was also illustrated in
figure 6 of "Muscles". These motoneurons are frequently called
fusimotor neurons. All
motoneurons belong to what we called the
motoneuron level of spinal neural network
organization in Part I of this tech brief.
3. Interneurons (INs), which are in turn divided into three subcategories as
Segmental interneurons have relatively short axons and project their outputs only to other interneurons or motoneurons in the same vertebra segment and sometimes to the neighboring one or two vertebra segments on either side of the segment wherein they reside. Most segmental interneurons are named after the group of afferent signals that predominantly affect them, e.g. "group II INs". Propriospinal tract INs are in many ways similar to segmental INs but have long axons and can project their outputs over most of the length of the spinal cord. Their principal role seems to be in coordinating groups of synergist muscles during voluntary movement. Ascending tract interneurons project their outputs to the brain. They are the primary feedback path for information sent to the higher motor centers in the brain.
1 E. Jankowska, M.H. Gladden, and J. Czarkowska, "Modulation of responses of feline g-motoneurons by
noradrenaline, tizanidine, and clonidine",
J. Physiol. (1998)
512.2: 521-531.
SSMS II: Neurons
Figure 1: Layout of the spinal cord gray matter. The spinal cord is laterally symmetric about the median
line. Its anatomy is described in terms of 10 laminae, illustrated above for the left side of the cord. For our
purposes we can regard laminae I – VI as comprising the dorsal horn, and laminae VII – X as comprising
the ventral horn. (In fact, lamina VI is a kind of transition zone between the dorsal and ventral horns).
Neuron function can often be guessed at by knowing which laminae contain that class of neurons. The
right-hand side of the cord illustrates the relative position of four important general types of spinal neurons.
Note the extensive arboration of the motoneuron dendrites. The top right-hand side of the figure illustrates
the location of the Ia afferent sensory neurons in the dorsal root ganglion and the path taken by this group
of spindle afferents into the spinal cord proper. Note that the Ia pathway projects all the way into the motor
nuclei (where the motoneurons are located). Note, too, that the Ia afferent projects into the dorsal column in
the white matter, and thereby sends afferent signals to other vertebra segments. Other types of afferents do
this as well. We will discuss the other three illustrated interneurons in the text.
The function of, or at least the signal pathways processed by, an interneuron can often be
guessed at initially by where it is located within the spinal cord. Figure 1 illustrates the anatomical organization of the gray matter of the spinal cord. There are ten regions ("laminae") identified by the anatomists. Laminae I to V comprise the dorsal horn. Laminae VII to X comprise the ventral horn. Lamina VI is a kind of transition zone, but for our purposes we will regard it as part of the dorsal horn.
While several of the major classes of interneurons are well-studied, it remains true today that
the majority of interneurons in the spinal cord are still poorly understood. Many of the names assigned to specific types of interneurons actually designate an entire genus of neurons whose main common feature is the type of afferent signals that predominate their actions. In most cases, interneurons process a variety of afferent signals (especially in the propriospinal or PPS level of our neural network topology). Hence, a "group II IN" may also process group Ia and/ or group Ib afferents, joint and cutaneous afferents, etc. as part of the signal processing it does on group II afferents.
SSMS II: Neurons
For purposes of network classification, we will define the motoneuron level (MNL) of our
neural topology as the level that consists of all the motoneurons, two types of segmental
interneurons known as the
Ia-IN and the
Ib-IN, a class of segmental INs we will call IIVH-INs,
and another type of interneuron known as the
Renshaw cell (RC). All other types of interneurons
I assign to the propriospinal level (PPSL) of our neural network topology. The afferent neurons
and supraspinal neurons (located in the brain) that project axons carrying descending command
signals down the spinal cord we will regard as
input layer neurons (ILNs).
A
pathway is defined as a sequence of neurons in series processing a signal from a specified
source neuron (usually an afferent sensory neuron) to a specified destination neuron (often, but
not always, a motoneuron). The same neuron may be, and often is, a part of a number of different
pathways. A pathway is called
monosynaptic if the source neuron projects directly to the
destination neuron. It is called
disynaptic if the source neuron signal reaches the destination
neuron after passing through one intermediate interneuron. Such a neuron, in standard neural
network theory parlance, is called a "hidden layer" neuron. A pathway is
trisynaptic if the signal
passes through two interneurons on its way from source to destination. The term
polysynaptic is
used for pathways known to not be monosynaptic but where it is uncertain how many hidden
layers are involved. (The prefix "poly-" means "many"). The term
oligosynaptic is used when it
is known that there are few, if any, hidden layers but the number of hidden layers is not actually
known. (The prefix "oligo-" means "few"; an oligosynaptic pathway might be monosynaptic, but
it would not be known for sure if it was really monosynaptic). A pathway is said to exert
excitatory action if it produces excitatory post synaptic potentials (EPSPs) in the destination
neuron. A pathway is said to exert
inhibitory action if it either produces inhibitory postsynaptic
potentials (IPSPs) in the destination neuron or if it produces
presynaptic inhibition that blocks
action potentials from reaching the destination neuron.
The pathways define the topology of the
neural network.
The a
-Motoneurons and Their Pathways
We will start with the principal output neurons of the SSMS, the a-MNs. These come in a
progression of sizes, from small to very large. Small a-MNs are the most easily excitable, are
typically the first to fire during a movement, and output to S-type skeleto-muscle fibers. The ease of excitability lessens as a-MNs increase in size from S-type to FR-type to FF-type. As we stated
in the "Muscles" tech brief, a single a-MN can innervate a large number of extrafusal fibers, and
this ensemble of fibers is called its
motor unit (MU). As we also mentioned, the SSMS controls
the amount of force and effort exerted by the muscles by
recruiting motor units as needed,
thereby increasing the number of contracting extrafusal fibers in the muscle. Recruitment simply
means that more a-MNs are activated, thereby activating their muscle units in parallel with the
ones activated earlier.
Recruitment invariably follows the same progression. First S-type motor units are activated
and recruited; they are followed later by FR-type MUs; finally, when a great deal of force is required, the FF-MUs are recruited. The reverse order is followed in de-recruitment (reduction of the muscle force being applied). It is widely believed that the relative sizes of the a-MNs, which
correlates inversely to the ease with which they can be excited into firing, is one of the mechanisms that govern MU recruitment.
Although there is significant variation from one a-MN to another in what signal pathways
converge on it, all a-MNs seem to follow a common scheme insofar as what signals it receives.
The a-MN input pathways are as follows.
SSMS II: Neurons
Homonymous and Heteronymous Monosynaptic Ia Pathways. First, every a-MN whose MU
is part of the same muscle receives monosynaptic excitatory inputs from group Ia afferents
coming out of that muscle. Feedback of an afferent signal from a muscle to the neurons that
control that muscle is called
homonymous feedback, and the afferents are called homonymous
afferents. It is known that more than 90%, and most probably 100% of a muscle's group Ia
afferents contact every a-MN of that muscle (which are therefore called the homonymous a-MNs
of that pathway).hese very same Ia afferents also make monosynaptic excitatory contact with a-MNs in other synergist muscles, but with less coverage (i.e. not every synergist a-MN is
contacted by a group Ia afferent from the agonist muscle). Close synergists, e.g. the lateral
gastrocnemius when the medial gastrocnemius is the agonist, may receive on the order of about
65% coverage from group Ia afferents coming to it from the agonist. Ia afferents coming in from
other muscles are called
heteronymous pathways.
EPSPs induced in the MN from individual homonymous Ia fibers are small, averaging about
102 mV per action potential (AP) pulse. Heteronymous EPSPs from a single Ia fiber are slightly
smaller, averaging about 82.5 mV. EPSP distributions are illustrated in Figure 2.2 A typical MN
receives inputs from an average of about 60 Ia afferents, which combined produce EPSPs of a few millivolts.
Recall that Ia sensory neurons are "velocity sensors." Thus, firing a MN and producing a
muscle twitch will tend to activate Ia pathways, which then increase the Ia afferent excitation of the a-MN, which then increases the twitch (if the MN fires as a result), etc. We can see that this
pathway is capable of constituting a positive-feedback system to the MNs. This is what one might expect from a
reflex, but it also places obvious limits upon the "loop gain" (feedback synaptic weights) that can be tolerated in the system for stability reasons. Recall, too, that g-MNs affect the
Figure 2: Histograms showing distribution of EPSP amplitudes for homonymous and heteronymous
motoneurons. The average for homonymous MNs is about 102 mV. For heteronymous MNs the average is
about 82.5 mV. A typical a-MN receives an average of about 60 Ia afferents, and simultaneous AP inputs
from each of these evokes an EPSP of around 6 mV on homonymous MNs, and about 3 mV on
heteronymous MNs. Data and graph are taken from Mendell & Henneman2.
2 L.M. Mendell and E. Henneman, "Terminals of single Ia fibers: location, density, and distribution within
a pool of 300 homonymous motoneurons",
J. Neurophysiol. (1971)
34: 171-187.
SSMS II: Neurons
response of muscle spindle sensors, and so fusimotor signaling to these motoneurons also enters in to the "control system" picture of this afferent pathway.
Reciprocal Ia Inhibition Pathways. The neurotransmitter (NTX) released by Ia afferents is
believed to be glutamate (glu)lu is exclusively an excitatory NTX and therefore
all
monosynaptic connections from Ia afferents are excitatory. Nonetheless, it is also a fact that
signal pathways from Ia afferents also produce IPSPs in MNs. The only way this is possible is if
the inhibitory pathway from Ia afferent to MN is at least disynaptic with the last-order IN being
an inhibitory IN.here are three distinct classes of inhibitory Ia pathways to MNs, called the
recurrent (also called
autogenetic),
reciprocal, and the
non-reciprocal pathways. The word
"recurrent" means that the IPSP was stimulated by the firing of the MN that is being inhibited.
Reciprocal means the inhibition was due to the firing of a different MN (usually an antagonist or
a synergist of an antagonist). Non-reciprocal means that synergists are the target of the inhibition.
We describe now the reciprocal Ia inhibitory input to the MN.
Reciprocal inhibition is a key factor in myotatic reflexes. The firing of, say, a flexor MN
leads to the inhibition of the firing of MNs in its antagonist extensor muscle. Inhibitory inputs to MNs through a Ia reciprocal inhibition pathway are mediated by class of last-order INs called "Ia inhibitory interneurons" (Ia-INs). These INs are the only interneurons with a dominating peripheral inpu Ia afferents.l have more to say about Ia-INs in Part III). MNs receive monosynaptic inhibitory inputs from Ia-INs. A typical MN will receive inputs from on the order of about 70 Ia-INs and most have more than 10 Ia-IN inputs. The inputs synapse to the soma and proximal dendrites of the MN (cf. our tech brief "Dendritic computation in multi-compartment neurons). The NTX is glycine (an inhibitory NTX), and the ionotropic synaptic input currente chloride. Single fiber APs evoke IPSPs in the range from 8 mV to 220 mV,
which are from 10 to 200 times smaller than the maximum IPSPs evoked in a-MNs via this
pathway
This pathway from Ia afferent to motoneuron is disynaptic.
Non-reciprocal Group I Inhibitory Pathways. Non-reciprocal inhibition of MNs from group
I afferents (i.e. from Ia and Ib spindle afferents) is another important inhibitory pathway. At present it is believed that this pathway is mediated by a last-order inhibitory interneuron known as "the Ib inhibitory interneuron" (Ib-IN). This IN was so-named because at the time of its discovery it was thought that only Ib afferents (Golgi tendon organ afferents) contributed to peripheral excitation of this IN (which, of course, would be a peripheral inhibition of the MN).
3 R.E. Burke, "Spinal cord: ventral horn", in
The Synaptic Organization of the Brain, G.M. Shepherd (ed.), 4th edition, NY: Oxford University Press, 1998, pp. 77-120.
4 A
last-order interneuron is a hidden-layer IN that directly drives the destination neuron. It is, therefore,
an IN in the final hidden layer. A
first-order interneuron is an IN in the first hidden layer, driven directly
by the input neuron.
5 An input is
peripheral if it comes in from the peripheral nervous system, i.e. if it comes from muscle,
joint, and cutaneous sensory neurons via the dorsal root. An input is
supraspinal if it comes down from the
brain. An IN can have both a dominate peripheral input and a dominant supraspinal input. Dominant means
that the input exerts the strongest direct effect on the IN of all the IN's inputs of that class. Thus "dominant
Ia input" means the Ia afferents have more affect on the IN than the Ib, II, III, etc. peripheral inputs, each
considered individually.
6 E. Jankowska, "Interneuronal relay in spinal pathways from proprioceptors,"
Prog. Neurobiol. (1992)
38: 335-378,
7 R. Wells, "Modulation channels in biomimic artificial neurons,"
Proc. 28th Ann. Conf. Indus. Electron. IECON02, Nov. 5-8, Seville, Spain, pp. 3209-3214.
8 E. Jankowska and W.J. Roberts, "Synaptic action of single interneurons mediating reciprocal Ia inhibition
of motoneurones",
J. Physiol. (1972)
222: 623-642.
SSMS II: Neurons
It is now known that Ia afferents have an inhibitory pathway to MNs through the Ib-IN.
The
shortest pathway in this case is disynaptic (Ia afferent to Ib-IN to MN), but it is also known
that trisynaptic pathways exist (Ia afferent to a first-order IN to the Ib-IN to the MN). In some
cases, particularly the disynaptic ones, the inhibition is autogenetic (i.e. the Ia afferents are from
the muscle of the MN being inhibited). In general, inhibition from Ib afferents converging on the
Ib-IN are stronger than those associated with the Ia afferents. Ia afferents account for only about
10% of the IPSPs invoked in MNs.
The Ib afferent pathways are both disynaptic and
trisynaptic (from Ib afferent to MN). For this reason, it is hypothesized that the primary effect of
Ia afferents in this pathway is to support inhibition by other afferents (notably the Ib afferent from
the Golgi tendon organ) by raising the excitation level of the Ib-IN without actually producing
very much firing activity by themselves. Like the Ia afferents, the Ib afferents can also be
autogenetic. However, for both types of afferents non-autogenetic inhibition from synergist and/
or antagonist muscles do exist.his pathway is most commonly known as the Ia/Ib non-
reciprocal inhibitory pathway. There appears to be no clear pattern of convergence of these
afferents on Ib-INs insofar as any "dominant muscle" source of origin of the afferents is
concer
Recurrent Inhibition. Arguably one of the most important sources of inhibition of MNs is a
pathway running
from the MN axon back to the MN itself. The neurotransmitter for
motoneuron axons is acetylcholine (ACh), which is an excitatory NTX. Therefore, this recurrent
inhibitory pathway must be mediated by an inhibitory IN. This IN is known as the
Renshaw cell (RC). The MN axon extrudes a collateral fiber to the RC, which in turn makes an inhibitory
synapse with the MN. This will be discussed in more detail when we talk about Renshaw cells
and motoneuron-level circuit organization.
Inhibition and Excitation by Descending Supraspinal Tracts. Descending supraspinal inputs
from the corticospinal, vestibulospinal, rubrospinal, and reticulospinal tracts all have polysynaptic inhibitory pathways to the MNs. However, these pathways pass through the Ia-IN en route to the MN, and so we will discuss them when we discuss the Ia-IN and when we discuss the PPS level of the spinal neural network system. In addition, primates (including humans) are thought to be unique in having monosynaptic excitatory connections to a-MNs from corticospinal and rubro-
spinal descending tracts. Corticospinal EPSPs are smaller than EPSPs due to Ia afferents, and they exhibit facilitation from tetanic firing (cf. "Synaptic weight modulation and adaptation part I" tech brief). Some vestibulospinal tract fibers also make monosynaptic excitatory connection with a-MNs, and these connections behave like group Ia connections.3 Rubrospinal tract fibers
connect with a-MNs of distal extremities (e.g. fingers, toes) but the main role of the rubrospinal
tract seems to be movement fractionation, the capacity for independent, flexion-biased movements of the shoulders, elbow, and hand.
Excitation and Inhibition by Group II Afferents. The group II spindle afferent is the length-
sensing sensor in the muscle. There are both monosynaptic and disynaptic excitatory pathways from group II afferents to the MN. There is also an important trisynaptic inhibitory pathway from group II to the MN. The most important effects of group II afferents take place through the poly-synaptic pathways, which are mediated by group II interneurons. In part I we noted that group II
9 E.E. Fetz, E. Jankowska, T. Johannisson, and J. Lipski, "Autogenetic inhibition of motoneurones by
impulses in group Ia muscle spindle afferents",
J. Physiol. (1979)
293: 173-195.
10 P.J. Harrison and E. Jankowska, "Organization of input to the interneurones mediating group I non-
reciprocal inhibition of motoneurones in the cat",
J. Physiol. (1985)
361: 403-418.
11 V.B. Brooks,
The Neural Basis of Motor Control, chap. 5, NY: Oxford University Press, 1986.
SSMS II: Neurons
afferents are most commonly associated with the group II reflex, which is thought to be involved in posture control. (Recall that group II muscle spindles detect stretch). However, the most important role for group II pathways is likely to be as control pathways by which the brain mediates motor commands during complex movements.ill discuss this in more detail when we talk about the PPS level of the network hierarchy.
Interneurons predominantly affected by group II afferents are called "group II INs", and it
seems to be the case that they come in two major forms: dorsal horn group II INs that do not project to motoneurons, and group II INs located in the ventral horn that do. Ventral horn group II INs are located in laminae VI and VII, and we will denote them as IIVH-INs to distinguish them from the dorsal horn INs (IIDH-INs). It also appears to be the case that there are two species of IIVH-INs: INs projecting excitatory signals to a-MNs (IIVH-EINs), and INs projecting inhibitory
signals to a-MNs (IIVH-IINs). The NTX used by IIVH-IINs is thought to be glycine, although it
has not been ruled out that some GABAergic INs might exist. The NTX used by IIVH-EINs has not been established so far as I know.6 The principal inhibitory pathways involving group II INs appear to be trisynaptic; the principal excitatory pathways through IIVH-EINs appear to be disynaptic. The IIVH-INs are the last-order interneurons in the group II afferent pathway to MNs, and we will regard them as input layer neurons in the MNL neural networks. Many IIVH-INs converge on a single a-MN.
As for the monosynaptic group II pathway, a-MNs receive inputs from about 50% of the
homonymous group II afferents and about 17 – 26% of the heteronymous group II afferents.The amplitudes of single-fiber EPSPs are roughly half that of the Ia afferents, and total EPSP evoked in the MN from simultaneous APs coming in from all converging monosynaptic group II afferents is roughly 25% of that of group Ia responses.t follows that although monosynaptic group II pathways are considerably weaker than group Ia monosynaptic pathways, they are not negligible. The role of this positive feedback pathway is a matter of some significant conjecture. Suggestions have included13: tonic excitation of anti-gravity musculatureaintenance of excitability level in motoneurons (i.e. a kind of "Q-point bias" in electronics terminology), minimization of oscillations in the muscle feedback control system (although I personally have a problem with viewing excitatory group II feedback as playing such a role; inhibitory group II feedback is, of course, another matter), or production of the tonic stretch reflex. (So far as the monosynaptic pathway is concerned, this reflex would show up as contraction of a muscle when the muscle is being stretched; refer to figure 13C in part I).
Flexor Reflex Afferent (FRA) Pathways. The flexor reflex afferents properly include
cutaneous, joint, and muscle group III and IV sensory signals. Prior to the discovery that group II afferents make monosynaptic contact with MNs, the group II afferents were also widely regarded as part of the "FRA system." However, since then inclusion of group II afferents in this grouping has been controversial. What seems to make the most sense is to exclude monosynaptic group II pathways from being included in the FRA system, and referring to the totality of FRA afferents
12 A. Lundberg, K. Malmgren, and E.D. Schomburg, "Reflex pathways from group II muscle afferents 3.
Secondary spindle afferents and the FRA: a new hypothesis",
Exp. Brain Res. (1987)
65: 294-306.
13 J.B. Munson, J.W. Fleshman, and G.W. Sypert, "Properties of single-fiber spindle group II EPSPs in
triceps surae motoneurons",
J. Neurophsyiol. (1980)
44: 713-725.
14 E.K. Stauffer, D.G.D. Watt, A. Taylor, R.M. Reinking, and D.G. Stuart, "Analysis of muscle receptor
connections by spike-triggered averaging. 2. Spindle group II afferents",
J. Neurophysiol. (1976)
39: 1393-
1402.
15 "Anti-gravity musculature" is the use of muscles to oppose the pull of gravity in maintaining body and limb position.
SSMS II: Neurons
and polysynaptic group II afferents as the "general reflex afferents" or GRA (a bit of terminology peace-making proposed by Nobel laureate Eric Kandel).
FRA pathways are polysynaptic, and there are a great many of them running in parallel to
one another through hidden layer INs of various depths. There are no monosynaptic FRA pathways to a-MNs, and the polysynaptic pathways include both excitatory and inhibitory
connections to the a-MNs.12 Perhaps less is known about the details of the FRA pathways than
about any other aspect of the SSMS. It appears to be the case that the FRA pathways to the a-
MNs always involve group II polysynaptic pathways and descending tract supraspinal signals as well. It is therefore appropriate that we postpone discussion of the FRA pathways for when we talk about the PPS level neural network topology. Let it be sufficient here merely to say that it is widely believed that control of voluntary movements is carried out by means of the GRA pathway. It is also worth noting that some FRA pathways involve the actions of group I afferents (both Ia and Ib), and the current leading hypothesis holds that these pathways involve local central pattern generators (CPGs) that are essential in the control of repetitive movements, such as walking, that involve complex coordination of the actions of many different muscles.
These seven groups of pathways constitute all the known types of pathways that
converge on a
-MNs. The GRA pathways (FRA plus polysynaptic group II pathways) and the
FRA pathways involving groups Ia and Ib afferents in CPG action will be regarded as exclusively
belonging to the PPSL neural networks. Monosynaptic pathways, pathways involving the
Renshaw cells, the Ia-INs, the Ib-INs, and the IIVH-INs will be regarded as belonging to the
motoneuron level (MNL) neural networks in our SSMS neural network hierarchy.
The g
-Motoneurons and Their Pathways
Owing to their small size and the difficulties involved in probing them, the network
pathways to the g-MNs are less well established than is the case for the a-MNs. g-MNs drive the
intrafusal muscle fibers, which do not participate directly in motion. However, contraction of these fibers profoundly affects the firing of groups Ia and II spindle neurons, and we have just seen the extent to which these afferents feed back to the skeletomuscle control system. The g-MN
might therefore be regarded as a sort of gain control element in the instrumentation of the skeleto-muscle system.
There are a number of important differences between the g-MNs and a-MNs aside from the
obvious difference that the former drive intrafusal fibers while the latter drive extrafusal fibers. Figure 3, taken from a paper by Brooksts an illustration from our "Muscles" tech brief. Of the intrafusal fibers, the chain fibers are the most rapid to contract and to relax, followed by bag 2 fibers, and then bag 1 fibers. The relative contraction and relaxation times for these fibers, setting the chain fiber as reference, are 1, 1.4, and 2.6 respectively. The primary spindle afferents, i.e. the group Ia afferents, are 100 times more sensitive to small changes in length than to large changes. In other words, this sensor is highly nonlinear. After a large change, however, the spindle sensitivity returns following a time lag of about 1 second. In addition, the bag 1 fiber exhibits mechanical creep, i.e. the tendency of the bag 1 fiber to return to its original length, thereby reducing its sensitivity. Bag 1 fibers are more elastic (less stiff) and less viscous than are the secondary spindle sites. This makes bag 1 a "dynamic" fiber, i.e. its sensory output more reliably senses velocity than it does length. (And this accounts for the designations of the motoneurons that drive it as "dynamic" MNs). In addition, the primary spindles are too slow to be
16 V.B. Brooks, "Cerebellar functions in motor control,"
Human Neurobiol. (1984)
2: 251-260.
SSMS II: Neurons
Figure 3: Muscle sense organs and their motoneuron connections.
of much use in sensing length during a movement. Bag 2 fibers, on the other hand, are more linear in their response to stretch, but also less sensitive.
Intrafusal fiber biasing by static g-MNs raises the overall position sensitivity of the spindle.
Biasing by dynamic g-MNs does not. Simultaneous activation of gs- and gd-MNs tends to provide
a more continuous gain control for the spindles during large length changes. On the other hand, when movements are large and velocities exceed about 0.2 muscle lengths per second, activity in the fusimotor neurons does not significantly alter spindle firing characteristics.16 The
g-MN properties and the sensory pathways feeding to them are adapted to deal with
these properties of intrafusal fibers. Appelberg et alte several prominent differences between g-MNs and a-MNs. Among them are:
1. Powerful inputs from groups Ia and Ib fibers are the prerogative of a-MNs;
2. High-threshold afferent (i.e. group III) inhibition seems to be absent from a-MNs but it is
present in g-MNs; 3. gd-MNs have their own specific descending pathway from the midbrain;
17 B. Appelberg, M. Hulliger, H. Johansson, and P. Sojka, "Actions on g-motoneurones elicited by electrical
stimulation of group III muscle afferent fibres in the hind limb of the cat,"
J. Physiol. (1983)
335: 275-292.
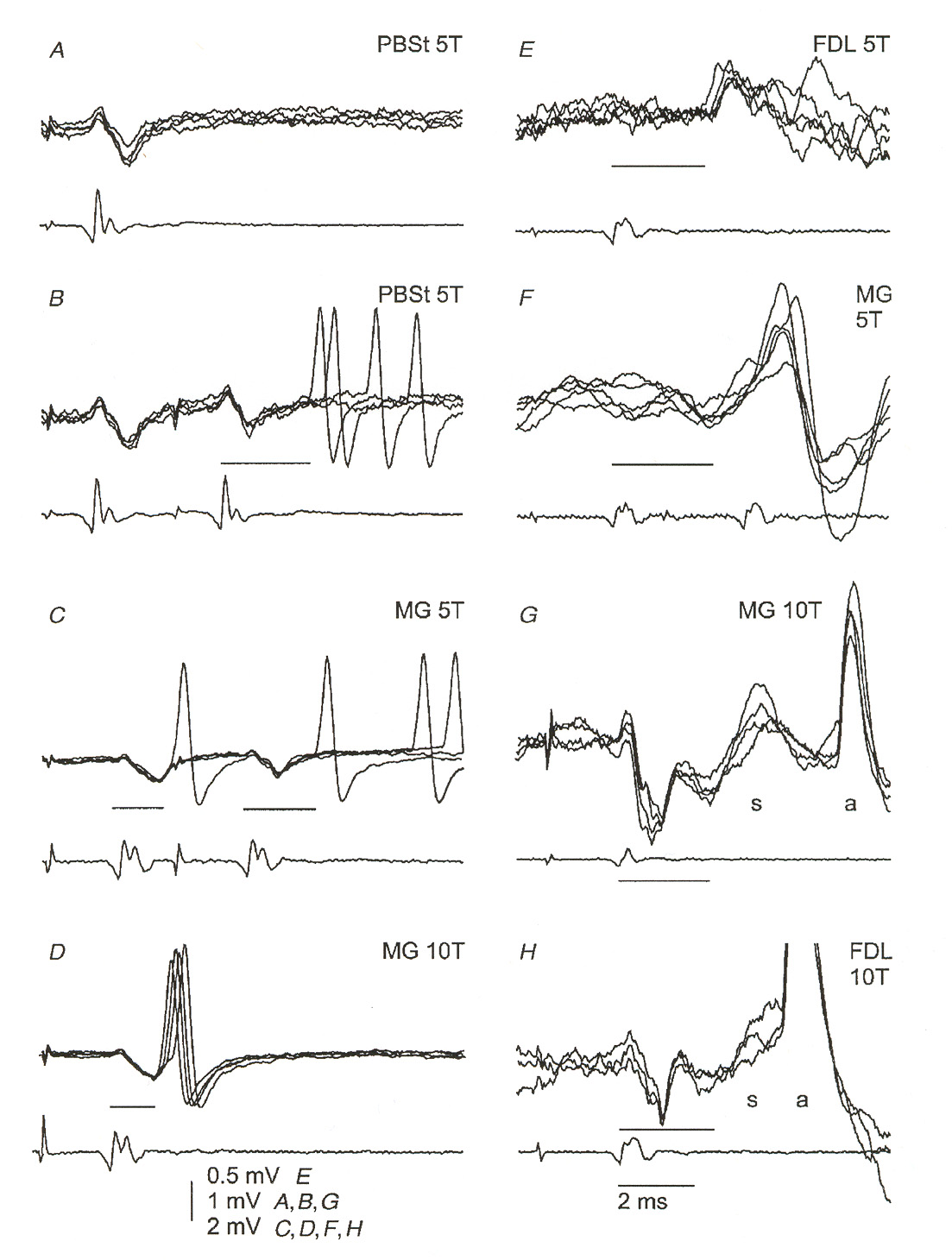
SSMS II: Neurons
4. Low-threshold cutaneous afferents act more powerfully on gd-MNs than on gs-MNs, and activity
patterns from high-threshold skin afferents to the g-MNs do not conform to the same patterns as
they do for a-MNs;
5. Joint receptors act more potently on g-MNs than on a-MNs.
Furthermore, the electrical activity of g-MNs differs significantly from that of the a-MNs.
An a-MN's membrane response to synaptic inputs conforms to the general characteristics of a
"leaky integrator". It has a relatively rapid rise time during EPSPs and a significantly slower fall time. Membrane response of g-MNs on the other hand more closely resembles the membrane
response of a classical Hodgkin-Huxley axon. Figure 4 illustrates some measured g-MN
membrane responses to EPSPsure 5 (also taken from [18]) illustrates two EPSPs evoked by
Figure 4: Measured EPSPs in g-motoneurons. Each figure shows several responses from the same
neuron overlapped on the oscilloscope trace. The labels identify specific neurons in the experimental animal and the amount of stimulus applied. The lighter traces below each figure were recorded at the surface of the spinal cord. Figure H illustrates a neuron responding with an action potential. Note the
characteristic dipulse response of the EPSPs. This same rising pulse followed by an undershooting minor
second pulse is very similar to the subthreshold response of a classical axon membrane.
18 M.H. Gladden, E. Jankowska, and J. Czarkowska-Bauch, "New observations on coupling between group
II muscle afferents and feline g-motoneurones," J. Physiol. (1998) 512.2: 507-520.
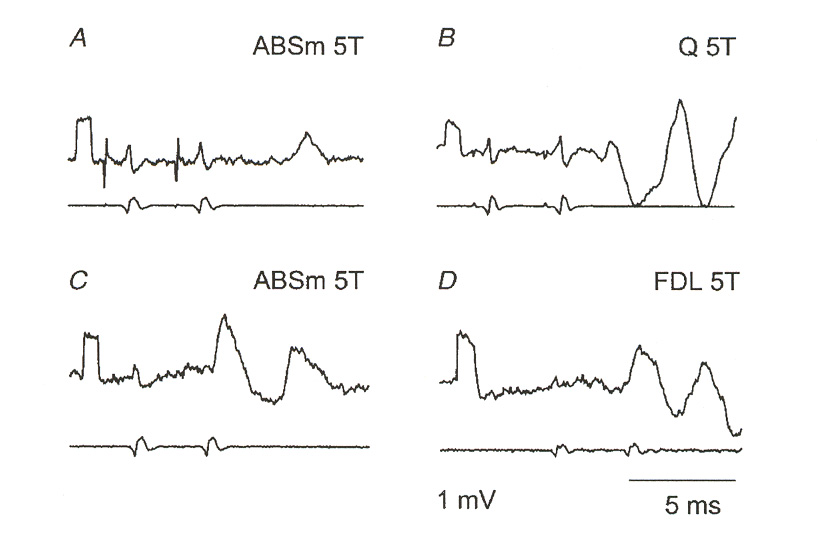
SSMS II: Neurons
Figure 5: g-motoneuron response to double stimulation below firing threshold. Figures C and D are
particularly good illustrations of the effect. Note the lower peak amplitude of the second EPSP compared to
the first. This behavior is found in subthreshold stimulation of Hodgkin-Huxley axons, where it is caused
by partial activation of voltage-gated potassium channels. This figure and the previous one illustrate the
interesting similarity between H-H axon membranes and the g-MN response.
a double stimulus. Note in this figure how the second EPSP has a lower amplitude than the first. This, too, is characteristic of an axon stimulated below its firing threshold.
This lack of any integrating action on the part of g-MNs is a behavior remarkably different
from the typical "leaky integrator" type of response found in most synaptically-excited neurons.
While the g-MN does "sum" its responses to synaptic excitations, it acts much more like a
threshold-detecting pulse generator or "one-shot". This simile is further enforced by the fact
that g-MNs do not appear to generate their own background tonic firing patterns, but instead their
tonic firing appears to be a response to inputs it receives from higher-level interneurons.
Let us now turn to the input pathways to the g-MN.
Group I Afferent Pathways. Unlike a-MNs, g-MNs show very little response to group I
afferents (Ia and Ib afferents). It is known that group I afferents do make both excitatory and inhibitory oligosynaptic connections with g-MNs. However, these group I responses are found in
only about 4% of the g-MNs in the motor pool. Of this small fraction, inhibitory responses are
four times more frequent than excitatory responses. All excitatory responses that have been observed are autogenetic, whereas inhibitory responses could be either autogenetic or heteronymous. EPSP amplitudes were about 2 mV in gs-MNs and about 4 mV in gd-MNs.
Although it is not known what pathway is followed by these afferents, it is my hypothesis that excitatory effects are likely to be homonymous monosynaptic Ia pathways (because it is known that Ia afferents heavily invade their own motor nuclei, and reported latencies seem to be most consistent with a short travel distance for the signal). It is likewise my hypothesis that inhibitory inputs are likely to be mediated through Ib-INs (because it is known that Ia-INs do not make projections to g-MNs, whereas Ib-INs are known to do so).
19 E. Jankowska, M.H. Gladden, and J. Czarkowska-Bauch, "Modulation of responses of feline g-
motoneurones by noradrenaline, tizanidine, and clonidine," J. Physiol. (1998) 512.2: 521-531.
20 B. Appelberg, M. Hulliger, H. Johansson, and P. Sojka, "Actions on g-motoneurones elicited by electrical
stimulation of group I muscle afferent fibres in the hind limb of the cat," J. Physiol. (1983) 335: 237-253.
SSMS II: Neurons
Be that as it may, group I afferents are generally thought to be simply too scarce to play any
significant role in either the excitation or the inhibition of g-MNs. In fact, most investigators take
it for granted that group I effects on g-MNs are entirely negligible. Perhaps this is so. It does seem
quite likely that they can play no significant role during voluntary movement, nor do they seem likely to have much of a role in myotatic reflexes. On the other hand, I am somewhat reluctant to believe that this pathway connection evolved to no purpose whatsoeverhaps they might have a role in recruitment of g-MNs during the first stages of movements. I have never seen
anyone discussing whether the recruitment property of a-MNs also applies to g-MNs. However,
during the first stages of a movement group II spindle feedback (length-sensing signals) is very weak, and perhaps it might be the case that a small amount of group I feedback to the g-MN
motor pool could be useful in a brief "startup phase" of movement control. On the other hand, it might also be the case that b-MNs could supply whatever intrafusal fiber excitation might be
required to provide an initial bias to the intrafusal sensors. The question is uninvestigated. For our
purposes, we should allow for some small percentage of group I feedback in our EC studies,
keeping an eye on whether or not this connection pathway is beneficial in any way.
Group II Afferent Pathways. Group II pathways, on the other hand, are known to not be
negligible. g-MNs are highly responsive to group II afferents, and both excitatory and inhibitory
signal pathways exist. All such pathways are oligosynaptic, and there are a great many technical difficulties that present challenges in determining the number of hidden layers of INs present in different pathways.18 One of these is the diversity of different signal propagation latencies along various neuronal and axonal pathways. As a consequence, a number of assumptions go into the calculation of signal latencies (and it is through the calculation of latency contributions by axonal fibers that investigators form their conclusions on whether a pathway is monosynaptic, disynaptic and so on).
Current thinking holds that excitatory monosynaptic group II pathways do exist.18 Unlike the
case with monosynaptic group I pathways discussed above, monosynaptic group II pathways appear to contact between 35% and 50% of the fusimotor neuron motor pool. Also unlike the group I case, these pathways come from both homonymous and from heteronymous muscles. Figures 6 and 7 illustrate a number of different sources for excitatory group II pathways as reported by Gladden et al.18 Figure 6 shows input source distributions found for one particular group of g-MNs in an extensor muscle (muscle "MG"). Figure 7 illustrates the same thing for
several different receptor muscles. The data reported in figure 7 illustrates that input convergence from different sources varies widely from one type of muscle to another. We may note that the data presented for the MG muscle appears to be inconsistent between figure 6 and figure 7. However, the data in figure 6 is based upon stimulation of EPSPs regardless of whether or not the EPSPs caused the g-MNs to fire, whereas in figure 7 the distributions depict pathways where the
afferents actually activated the g-MNs (i.e. caused them to fire action potentials).
The data in figures 6 and 7 does not discriminate between static and dynamic g-MNs.
Gladden et al. hold that no significant difference in distributions holds between static and dynamic MNs. However, they did not actually try to distinguish between these two types in their experiment, and so their opinion is not really based on solid data. An earlier work strongly disputes this opinion on what seems to me to be much more solid experimental grounds.
21 Such teleological considerations are not objectively valid grounds in science. It is a fact that many types of genetic mutations are survival-neutral. Fingerprints are one example. On the other hand, the question "what might this structure be good for?" are legitimate starting points for research.
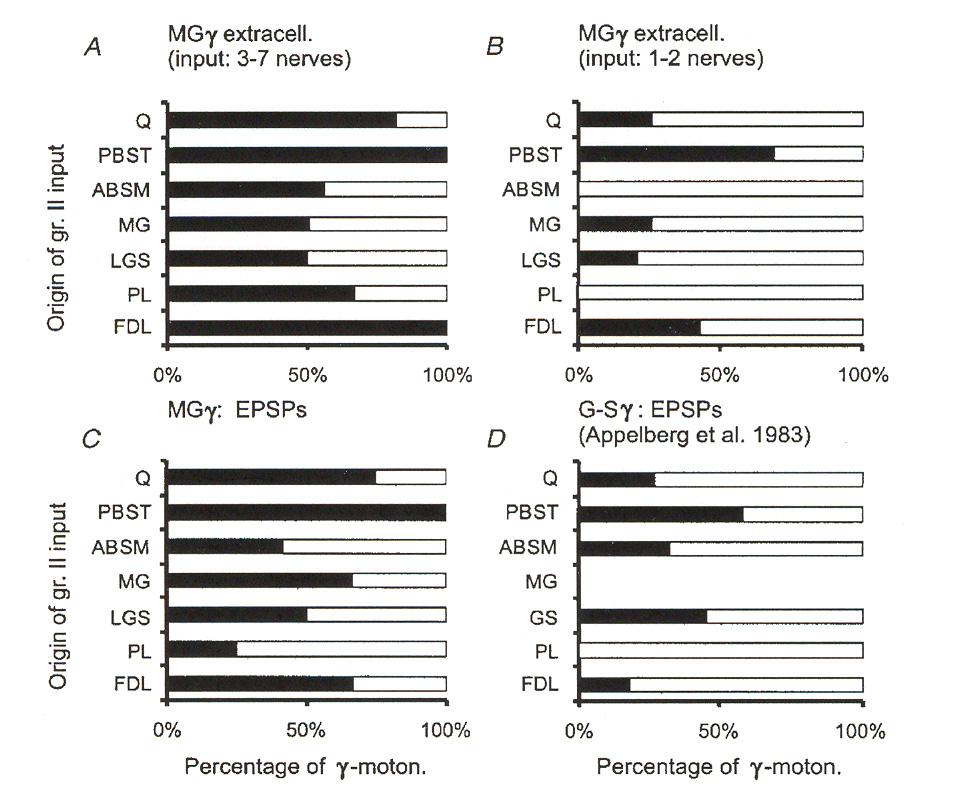
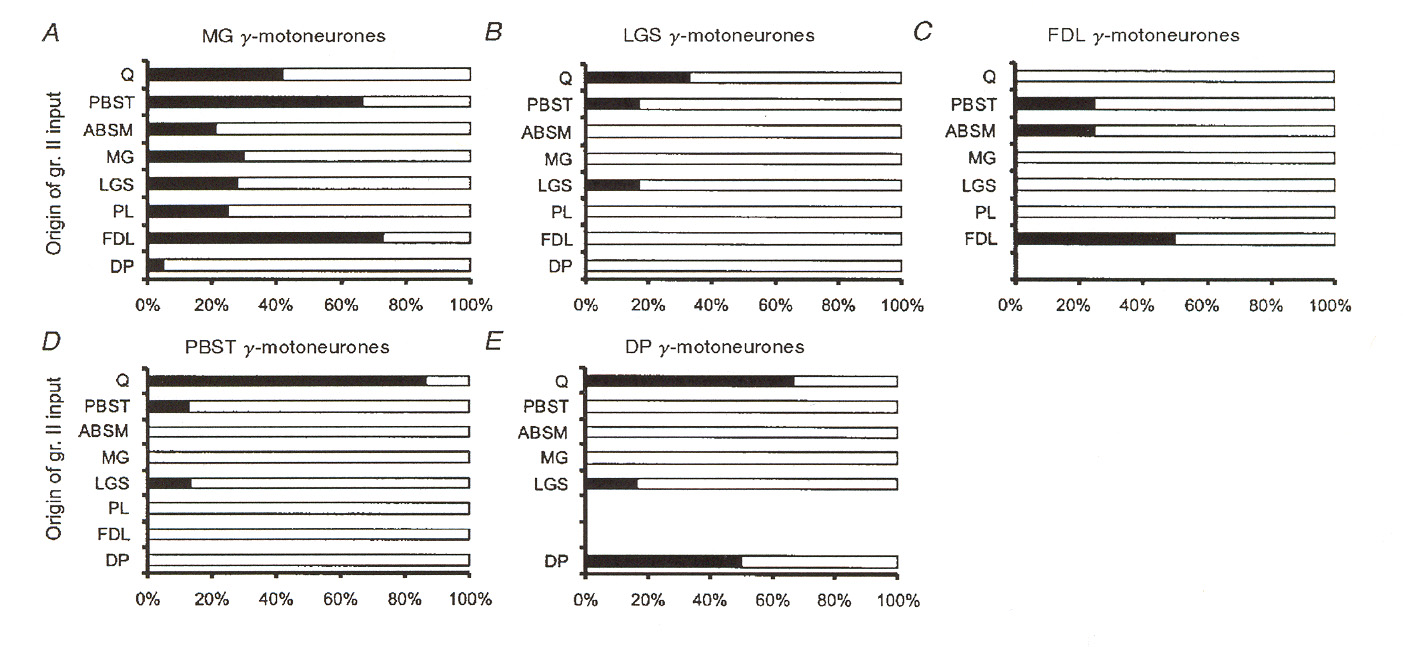
SSMS II: Neurons
Figure 6: Muscles of origin for excitatory group II afferents providing excitatory input to g-MNs in
an extensor muscle. The graphs plot percentage of neurons contacted by afferents from different muscles.
Sources include both synergist and antagonist muscles. Percentages refer to the percentage of cases where
EPSPs were observed in the group of tested MNs. A and B depict results for two populations of g-MNs
responding to 3 or more nerves or only 1-2 nerves. C is a pooled result for the entire test population of g-
MNs. D is data taken from the results of a different team of investigators. Muscle PBST is the flexor
antagonist of the MG muscle under test. C and D were based on intracellular recording (which is a more
sensitive way to detect post synaptic potentials). A and B are based on extracellular recording (which is a
less sensitive way to detect post synaptic potentials).
Figure 7: Muscles of origin for group II afferents providing input to different populations of g-MNs.
SSMS II: Neurons
In an earlier work Appelberg et alted that group II pathway distributions favored
dynamic g-MNs. Since this work actually made experimental distinction between static and
dynamic g-MNs, I tend to take their findings more seriously than those of Gladden et al. despite
the fact that Appelberg et al. apparently missed recognizing that some excitatory group II pathways are monosynaptic.
Group II excitation outweighs group II inhibition in g-MNs, but in this there is a significant
difference between extensor muscles and flexor muscles. Excitation outweighs inhibition in flexor muscles by a clear margin, but in extensors excitation only marginally outweighs inhibition. In analyzing different pathways to g-MNs Appelberg et al. categorized the signals they
were seeing at the g-MNs into four categories:
1. Pure group II action (MN input signals were clearly of group II origin only;
2. Predominant group II action (some group III signals were contributing to the action);
3. Undecided group II/III action (both group II and group III afferents were present);
4. Predominant group III action (group III afferents clearly outweighed group II).
Figure 8 illustrates the percentages of excitatory and inhibitory group II observations recorded for a flexor g-MN pool (p.b.s.t. cells) and an extensor g-MN motor pool (g.s. cells). The data is
blocked out according to whether the MNs were gs-MNs or gd-MNs. The parenthetical numbers in
Figure 8: Type and incidence of group II muscle reflex actions elicited in flexor and extensor
motoneurons by electrical single-shock excitation. The excitation data includes some cases where both
an excitatory and an inhibitory response occurred together. For that reason some percentages may add up to
more than 100. The %-inhibitory-only instances is given by 100 – excitatory percentage – no response
percentage. The figure is pooled data from all experimental observations and so does not illustrate the
distribution of variations due to different afferent sources. "D" stands for gd-MNs, "S" stands for gs-MNs.
22 B. Appelberg, M. Hulliger, H. Johansson, and P. Sojka, "Actions on g-motoneurones elicited by electrical
stimulation of group II muscle afferent fibres in the hind limb of the cat," J. Physiol. (1983) 335: 255-273.
SSMS II: Neurons
Figure 9: Relative frequency of group II reflexes from various muscle nerves on flexor (p.b.s.t.) and
extensor (g.s.) gamma motoneurons. P.b.s.t., D.p., S.d.p., G.s., Q., and F.d.l. are names for various
muscles. Percentages are expressed as percent of total number of observations and therefore give an
indication of the variations in afferent source frequencies. D denotes dynamic, S denotes static gamma
the graphs (e.g. the "71" in the upper left-hand column) give the number of observations for that column of data (excitation, inhibition, no response). Relative frequencies of group II reflexes according to afferent source for the static and dynamic MNs is illustrated in Figure 9. This data demonstrates that afferent source distributions are not homogeneous among the different source muscle nerves extending to the muscle under test. Note that excitation in the flexors (p.b.s.t.) is much more common than is inhibition. In the extensors (g.s.) excitation occurs only slightly more than does inhibition. Inhibition from antagonist muscles and some functional synergist muscles outweighs excitation in the extensor muscle.
The receptive territories (i.e. the number of different source muscles from whence came the
group II afferents) was very wide for individual g-MN cells. Reflex effects were elicited from a
variety of different muscle nerves as well as from a variety of skin and joint afferent fibers. This is illustrated below in Figure 10 for individual flexor g-MN cells and in Figure 11 for individual
extensor g-MN cells. Reflex action from autogenetic, synergistic, and even antagonistic muscle
nerves is frequent. However, neither autogenetic nor antagonist reflex effects are dominant. One
use we can make of these distribution properties in our EC work might be to establish
statistical distribution parametrics for neural network connectivities.
Determination of the number of hidden layers in these afferent pathways is difficult owing to
the relatively large variances seen in pulse latencies in the experimental animal. Figure 12 illustrates measured latency histograms seen for the dynamic and static motoneurons. This histogram is pooled data, but it nonetheless demonstrates oligosynaptic connectivity. The shortest excitatory pathway is monosynaptic, but disynaptic excitatory pathways are also in evidence. The shortest inhibitory pathway seems to be disynaptic but evidence of trisynaptic inhibitory pathways is also suggested.
SSMS II: Neurons
Figure 10: Input source distribution for group II reflex responses in individual g-MNs in a flexor
muscle. The key in the figure defines which of the four categories of afferent activity is depicted in each
box. The key also notes how to distinguish excitatory from inhibitory actions. The input abbreviations
across the top of the chart identify the different source muscle nerves. The numbers in the left-most column
identify individual g-MNs. Joint and skin afferent responses are also depicted. A: dynamic motoneuron
cells; B: static motoneuron cells.
The interneurons mediating the disynaptic and trisynaptic pathway have not been identified
(so far as I know), but they are believed to be group II INs. Evidence suggests that the IN in the
excitatory pathway is co-excited by group Ia afferents. Whether or not this is the IIVH-EIN
we discussed earlier in connection with the a-MNs is not known.
The IN mediating the inhibitory pathway is also not yet known. However, this IN could not
be the Ia-IN discussed earlier since this IN does not project to gamma motoneurons. It might possibly be the Ib-IN or it might be the inhibitory group II IN, i.e. IIVH-IIN. It
is known that IIVH-EINs project mainly to flexors whereas IIVH-IINs project mainly to
extensors. Such a distribution would be consistent with the results reported by Appelberg et al. above. Thus, while not ruling out other possibilities (including an entirely new class of group II INs dedicated to the fusimotor circuit pathway), it seems to be reasonable to suppose for now that IIVH-INs are the most likely candidate INs.
SSMS II: Neurons
Figure 11: Input source distribution for group II reflex responses in individual g-MNs in an extensor
muscle. The key in the figure is the same as for figure 10.
Figure 12: Measured group II signal latencies.
SSMS II: Neurons
Group III Afferent Pathways. In addition to high responsiveness to group II afferent
pathways, g-MNs also exhibit high responsiveness to group III muscle afferent pathways17. Group
III muscle afferents were discussed briefly in part I. While group III afferents are often and widely regarded as fast pain nociceptors, it is also known that group III fiber endings are pressure receptorser the past several years, an increasing number of neurobiologists have come to suspect that their pressure-sensing function is likely to be involved in the control and execution of voluntary movements. It is usually the case that extensor a-MNs are inhibited and flexor a-MNs
are excited by activity in group III muscle afferents17, which fall into the class of high-threshold
afferents. Appelberg et al. studied the effects of these afferents on g-MNs. They found that group
III afferents appear to act preferentially on gs-MNs, in distinction to the preferential action of
group II afferents on g-MNs. Both excitatory and inhibitory pathways are observed, and once
again excitatory reflex effects are more frequent than inhibitory effects. Flexors show a strong dominance of excitation over inhibition while extensors exhibit a weak prevalence of excitation over inhibition.
Appelberg et al. again categorized responses into the four groups discussed above for group
II afferent pathways. Figure 13 illustrates the breakdown of group III reflex actions for a flexor (p.b.s.t.) muscle and an extensor (g.s.) muscle. Figure 14 gives a side-by-side comparison of the group II and group III breakouts. It is easily evident in figure 14 that group II reflex effects (left columns of each pair in the figure) more prevalently affects dynamic motoneurons, while group III effects (right columns of each pair) more prevalently affects static motoneurons.
Figure 13: Type and incidence of group III muscle reflex action in flexors and extensors. The
conventions used in this figure are the same as those in figure 8. Excitatory dominance is readily apparent
for the flexor muscle (p.b.s.t.). Excitation in the extensor (g.s.) is only marginally more common than
inhibition. Note also the large percentages of "no effect" cases, which indicates that again group III afferent
innervation is selective (since input nerves eliciting no response would be nerves with pathways not
terminating on the group of motoneurons being tested). D = dynamic MN; S = static MN.
23 A.S. Paintal, "Functional analysis of group III afferent fibers of mammalian muscles", J. Physiol. (1960)
152: 250-270.
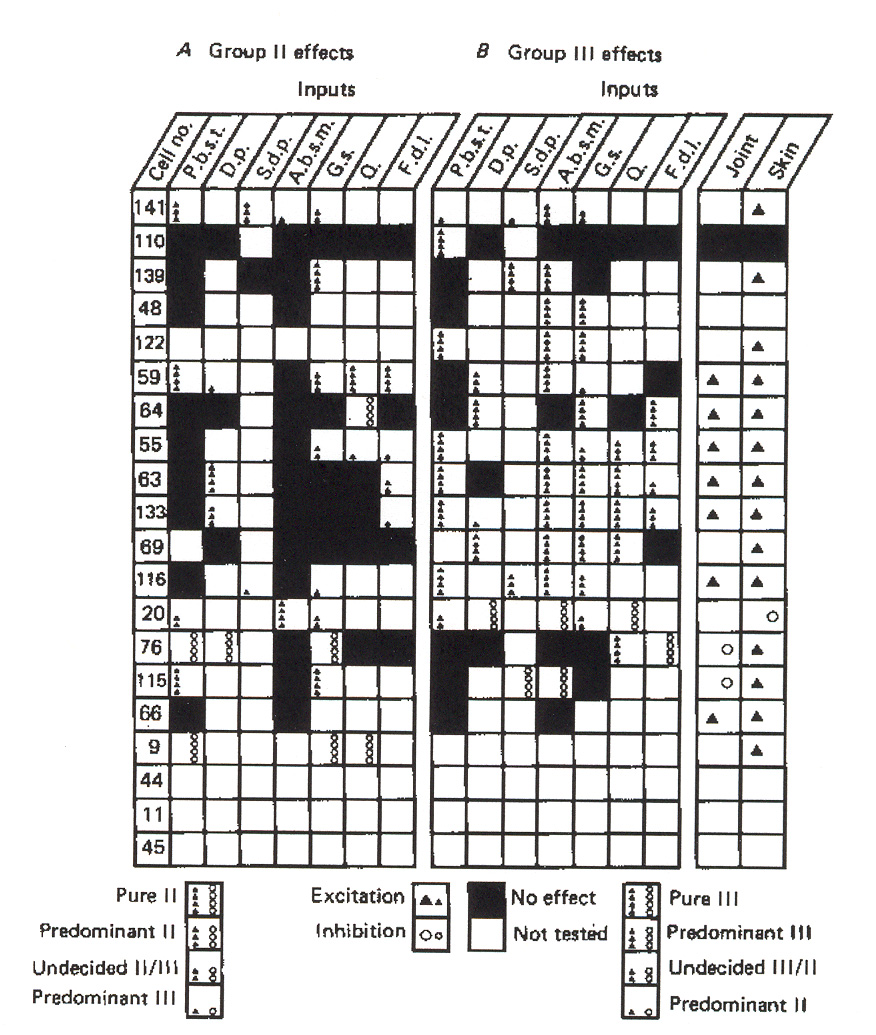
SSMS II: Neurons
Figure14: Comparison of group II and group III reflex effects for dynamic and static motoneurons.
Ambiguous (mixed II and III) test cases are excluded and so columns do not total up to 100%.
Figure 15: Groups II and III distributions to individual static g-MNs in a flexor muscle. The figure
also depicts joint and skin afferent inputs to these neurons. Symbols are explained in the key. The key
symbols also apply to figures 16 and 17.
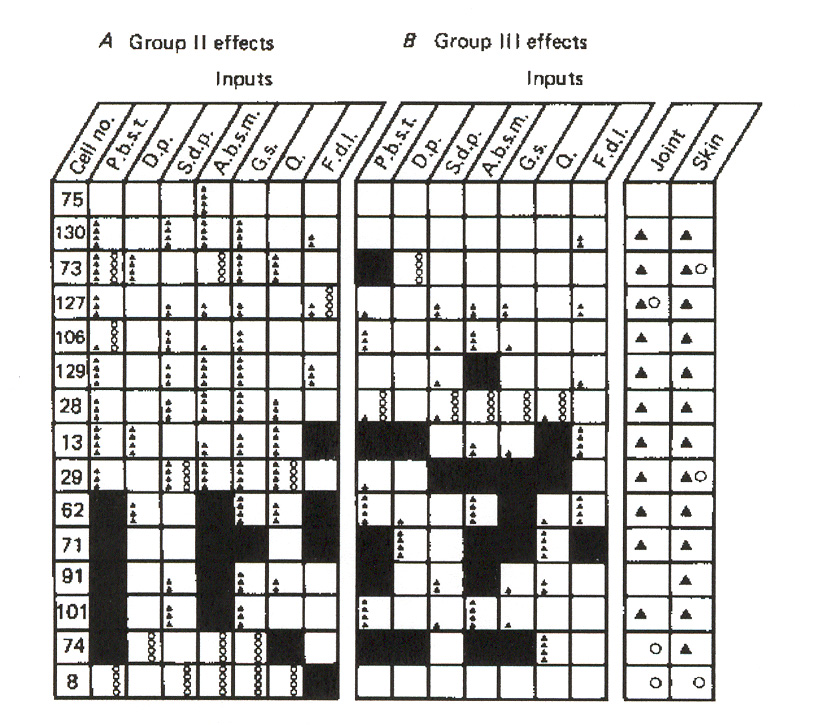
SSMS II: Neurons
Figure 15 provides a side-by-side comparison of group II and group III reflex actions on
individual static g-MNs in a flexor muscle as a function of afferent source. Figure 16 does the
same for the dynamic g-MNs, and figure 17 shows the comparison for dynamic extensor g-MNs.
The figures also exhibit joint and skin afferent responses observed. The key for all three figures is given in figure 15. Boxes in the figures shown as untested most likely imply that the specific neuron died before its test program could be completed.
FRA Pathways. The joint and skin afferents depicted in the figures above are part of the FRA
pathway system. Relatively little is known about the specific FRA pathways to g-MNs in terms of
the interneurons that are involved. It seems in general to be the case that these pathways are oligosynaptic, and that FRA afferents most likely do not make monosynaptic contact with g-MNs.
It is not known whether these FRAs share the same INs involved with pathways to the a-MNs, or
whether the group III afferents share INs in common with these neurons. There is some evidence that suggests that the FRA pathways to g-MNs are different from those of a-MNs. This would
seem to be reasonable since the data presented above illustrates that there is a great deal of variation among pathways to particular g-MNs.
Descending Supraspinal Afferents. It is beyond doubt that supraspinal descending tracts have
pathways to the g-MNs. Again, however, the details of these pathways are not well known. It is
believed that most of these pathways are polysynaptic rather than monosynaptic. However, it is not established whether or not these descending tracts contact last-order INs (forming a disynaptic pathway) or whether the pathway is trisynaptic or higher. There is some evidence that monosynaptic excitatory supraspinal pathways exist, and that these pathways may use the metabotropic neurotransmitter 5-HT (serotonin). It also appears to be the case that group II action on g-MNs can be depressed by the metabotropic neurotransmitter norepinephrine (NE; sometimes
called noradrenaline and symbolized as NA). It is thought that this takes place at the INs mediating group II pathways to the g-MNs.19
Figure 16: Groups II and III distributions to individual dynamic g-MNs in a flexor muscle. The figure
also depicts joint and skin afferent inputs to these neurons.
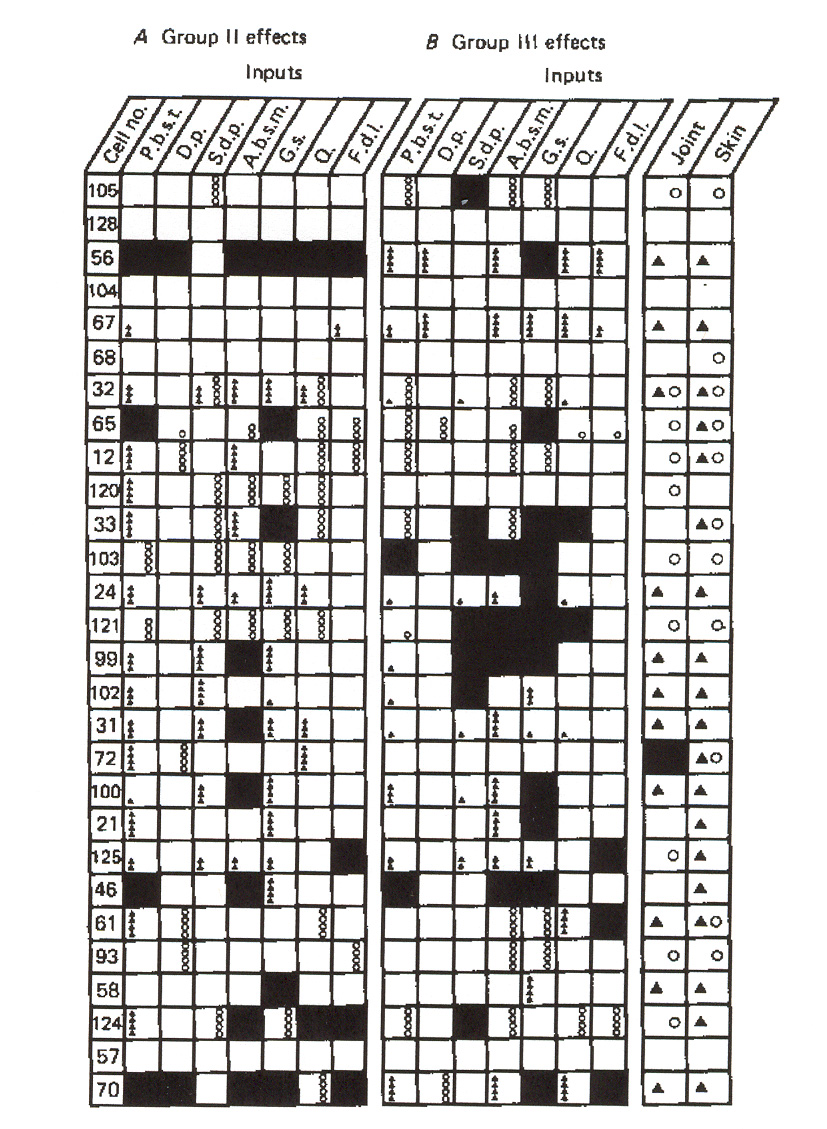
SSMS II: Neurons
Figure 17: Groups II and III distributions to individual dynamic g-MNs in an extensor muscle. The
figure also depicts joint and skin afferent inputs to these neurons.
a-g linkage. One fundamental hypothesis, accepted by many as well-established, is that of
"a-g linkage" in the overall motor control system. The essence of this hypothesis is this: In the
execution of a movement, stimulation by impulses in spindle afferents responding to the movement is not sufficient to establish control of the motion. Instead, co-activation of static g-
MNs is required to stimulate group II INs intervening in the control pathway in order to get the movement started. Once the movement is underway, excitation via FRA pathways is sufficient to maintain excitability of group II INs, by means of which movement control is effected. It seems pretty well established that feedback via group II afferents is the principal feedback control path during voluntary movements, and we recall that group II afferents arise from chain and bag 2 intrafusal fibers. These are the fibers excited by static g-MNs (and by b-MNs).
The central reasoning behind the a-g linkage hypothesis is based on what seems to be a clear
requirement that loop gain via group II afferents under conditions of passive muscle stretch must be low enough to avoid activation of motoneurons during the passive movement. Referring to figure 13C in part I, activation of an extensor muscle (i.e., contracting it) will passively stretch its antagonist flexor muscle. But there is a direct, excitatory path (i.e., a positive feedback loop) running from the group II sensors in the flexor through its a-MNs. If these group II afferents
were to succeed in firing the MNs, the flexor would contract, opposing the intended motion. As a specific example, what this means is that act of trying to straighten out your leg would produce a
SSMS II: Neurons
reaction by your calf and hamstring muscles that oppose contraction of your quadriceps muscles. This does not normally happen. But on the other hand, if group II afferent loop gain is low enough to prevent this from happening, then the voluntary motion (e.g. activation of movement by the quadriceps) could not be well controlled since it is known that group II pathways are critical during at least some phases of controlled movement.
One way out of this paradoxical situation is if the sensitivity of groups I and II afferents to
muscle stretch is controllable under descending commands from the brain. Lundberg et al.12 proposed the following hypothesis: Initiation of a voluntary movement depends primarily on supraspinal descending commands, and afferent signals play little or no part in motion control during this phase. Co-activation of static g-MNs by descending commands increases spindle
sensitivity ("raises the loop gain") in agonist control pathways (and inhibits it in antagonist pathways), thereby exciting the appropriate group II INs. (Recall that stimulating a g-MN
contracts the intrafusal fiber, which stretches the spindle). This increased activation of group II afferent neurons raises the excitability of group II INs, and the resulting positive feedback "kicks the movement into high gear" (so to speak). As the movement progresses, FRA afferents come into play and take over the servo control of the movement (in conjunction with descending tract commands). In effect, voluntary movement under this hypothesis follows a "gain scheduling" method, with the gain of different pathways changing at different phases of the movement, as instrumented by the various muscle and FRA sensors. It is indeed known for a fact that modulation of group Ib afferents (Golgi tendon organ afferents) takes place during voluntary movement, and that the modulation effects depend on limb position, forces acting upon the limb, and the type of movement (e.g. walking), and other FRA-related factorst is thought that FRA pathways acting upon CPGs mediate this modulation (i.e., it is thought that this is how the FRA pathways take over "at just the right time" when complex movements are underway).
Still, as attractive as the Lundberg hypothesis may be, the experimental evidence neither
confirms nor refutes its correctness. This is probably not a surprise because we are talking here about a very complicated system for which we still lack a complete neural "circuit diagram." For example, it seems to me to be a necessary requirement that the system be able to "turn down the gain" of the spindle afferents as well as to turn it up. It is known that under normal static conditions (e.g. standing posture) muscle spindle afferents exhibit tonic firing at a relatively low rate (compared to their firing rates under stretched or excited conditions). It is also known that complete relaxation (zero tension) of intrafusal fibers results in no firing of the spindle afferent neuron. Putting these together, we can say that under normal circumstances the intrafusal fibers are biased to some degree of reference tension. Since this can be done by b-MNs as well as by g-
MNs, actions mediated through either type of motoneuron can relax intrafusal fibers, thereby decreasing the sensitivity of muscle spindles.
Where does this leave us? The INs mediating g-MNs responses appear to belong to both the
PPS and MN levels of the neural network organization. Most of the excitatory IN pathways conveying group II afferent signals are likely to make both disynaptic and trisynaptic connections to the g-MNs, and the principal question is whether these same group II INs also mediate the
group II pathways to a-MNs. I suspect that we will find (through our evolution of the neural
connections) that some probably do and some probably don't. One important factor in this is likely to be the greater excitability of the g-MNs compared to the skeletomuscle motoneurons.
Another factor in determining the connectivity is likely to be that g-MNs display tonic
24 J.P. Gossard, R.M. Brownstone, I. Barajon, and H. Hultborn, "Transmission in a locomotor-related group
Ib pathway from hindlimb extensor muscles in the cat", Exp. Brain Res. (1994) 98: 213-228.
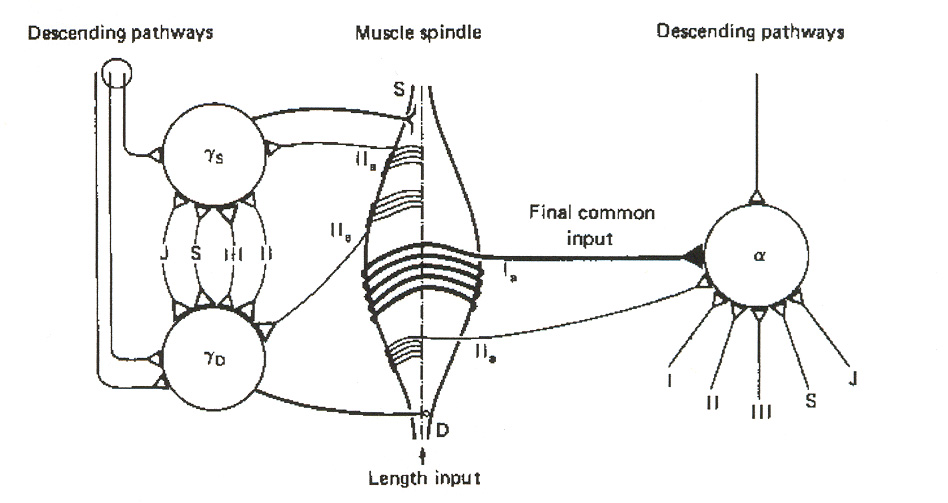
SSMS II: Neurons
background firing under non-movement conditions, whereas the a-MNs largely do not (with the
exception of those S-type motoneurons involved in maintaining posture). This tonic background firing is not a property of the g-MNs themselves; rather, it is due to tonic firing of some of the INs
that make synaptic connection with them. It is not known whether tonic firing of these INs is due to their own intrinsic properties or whether it is due to the action of pacemaker or CPG subnetworks.
It does seem to be well-established that group II excitatory pathways making monosynaptic
connection to g-MNs exist. Current thinking appears to favor the idea that these pathways would
not themselves typically suffice to fire g-MNs. However, they could, and probably do, provide
just enough extra excitability to determine which g-MNs will be the "first to fire."
Inhibitory group II pathways are likely to be mainly trisynaptic, although disynaptic
connection through inhibitory group II INs cannot be ruled out. Group III pathways are also likely to be of both the disynaptic and trisynaptic types. Other FRA afferents are likely to be mostly trisynaptic, and if so this would mean that these pathways contact first-order INs at the PPS level. I base this speculation on the fact that FRA pathways to a-MNs largely appear to be trisynaptic.
However, there is some significant evidence that disynaptic inhibitory FRA pathways to g-MNs
Appelberg et al. have proposed that the overall systematic role of g-MNs should be looked at
in terms of what they call the "final common input hypothesis. Figure 18 illustrates the basic idea. In this view, group I spindle afferents convey polymodal feedback information to the a-MNs and
to ascending tract pathways to the brain. Secondary spindle afferents (i.e. group II afferents) serve to constitute a link to the two types of g-MNs.
Figure 18: Final common input hypothesis. The figure does not attempt to illustrate INs in the signaling
pathways. J = joint afferent; S = skin afferent; III = group III afferent; II = heteronymous group II afferent;
IIa = autogenetic group II afferent. The intrafusal spindle fibers are deemed to act as integrators of the g-
MN signals, and to convey this integrated information as a "final common input" to a-MNs via group I and
group II afferents. The single lines in the figure denote multiple input signals, both excitatory and
SSMS II: Neurons
I will present a putative network for the connections to the g-MNs in a subsequent tech brief,
after I have discussed the various interneurons and their pathways. We will see there that most of
the pathway connections discussed here take place at the PPS level of the SSMS network.
Beta Motoneurons
To date my literature search has turned up little hard information on b-MNs. However, I
have not yet received all the papers I've ordered up on the subject, and I will provide an update on b-MN pathways later (assuming I can come up with some hard data on them). For now, our
best course seems to be to rely on the fact that b-MNs seem to be a peculiar sub-species of a-
MNs. It is widely thought that b-MNs receive the same kind of signals, and along similar
pathways, as the a-MNs. What makes them different is that they also drive intrafusal fibers. It
seems reasonable to suppose that bs-MNs have pathway connections similar to those of the FR-
type a-MNs, while bd-MNs are similar to S-type a-MNs.
Source: http://www.mrc.uidaho.edu/~rwells/techdocs/Spinal%20Sensorimotor%20System%20Part%20II.pdf
How to Put Data anD analytics to work in tHe era of HealtHcare reform anD analytics to work in tHe era of HealtHcare reform Demonstrated by Blue cross and Blue shield of north carolina How to Put Data anD analytics to work in tHe era of HealtHcare reform taBle of contents With a teradata enterprise data warehouse (eDW) at its core, blue cross and blue Shield of North carolina
Page 1 of 11 Jeff Gudin, Abel Gonzalez, Joon Lee Pain Management and Palliative Care, Englewood Hospital and Medical Center, Englewood, New Jersey, USACorrespondence to: Jeff Gudin, MD. Clinical Instructor, Anesthesiology, Icahn School of Medicine at Mount Sinai, Board Certified Pain Management, Anesthesiology, Palliative Care and Addiction Medicine; Director. Pain Management and Palliative Care, Englewood Hospital and Medical Center, 350 Engle St. Englewood, New Jersey 07631, USA. Email: [email protected]; Abel Gonzalez, MD. Department of Internal Medicine, Englewood Hospital and Medical Center, 350 Engle St, Englewood NJ 07631, USA. Email: [email protected]; Joon Lee, MD. Pain Management and Palliative Care, Englewood Hospital and Medical Center, 350 Engle St, Englewood NJ 07631, USA. Email: [email protected].